Deoxyschisandrin modulates synchronized Ca2+ oscillations and spontaneous synaptic transmission of cultured hippocampal neurons1
Introduction
The spontaneous and synchronous oscillations of intracellular Ca2+ concentrations ([Ca2+]i) are found in various types of neural tissues in vivo and in vitro, including the olfactory bulb[1], thalamus, hippocampus, and neocortex[2]. Together with spontaneous neurotransmitters release, these spontaneous and synchronous activities are believed to play a pivotal role during neuronal development[3], migration, information processing[4], and pathological events, such as epileptic seizures in the hippocampus[5]. In primary cultures of embryonic hippocampal neuronal networks, spontaneous and synchronous calcium oscillations are observed without external stimuli, resulting from periodic burst firing of action potentials through excitatory synaptic transmission[6]. This experimental model, which has been widely characterized in terms of synaptic development and functionality[7,8], also provides a convenient and accessible system to investigate the regulatory effects and underlying mechanisms for extracellular factors[6,9].
Schisandra chinensis, or “five-taste fruit”, is a Chinese herbal medicine which has been widely used for a long period of time. In traditional Chinese medical sciences, it is believed to have antioxidative, antiviral, antitumoral, and antisenile properties, and offers ischemia injury protection and cardioprotection. It is also used to enhance the functions of the liver and the immune system and to improve the neurological performance of healthy individuals under stressful conditions. Based on modern research, lignans extracted from Schisandra chinensis protect the mouse liver against carbon tetrachloride toxicity[10], enhance doxorubicin-induced apoptosis of cancer cells[11], and have an anti-HIV effect[12]. As for its effect on the central nervous system, it was reported that Schisandra chinensis and its effective composites have sedative, hypnotic, and antiepileptic effects, and improve intellectual performance. A previous study also showed that Schisandra chinensis protects primary cultures of rat cortical cells from glutamate-induced toxicity[13]. However, the exact cellular mechanisms of how Schisandra chinensis and its composites work on the central nervous system are less clear. In this paper, we chose Deoxyschisandrin (DS; molecular structure shown in Figure 1), which is known to be enriched in Schisandra chinensis[14] and is considered a major effective compound of the herb, to investigate its pharmacological effects on cultured hippocampal neurons. Further research evidence is needed to better understand the efficacies of Schisandra chinensis for clinical treatment and to expand the fields of application for this traditional Chinese medicine.
Materials and methods
Materials Dulbecco’s modified Eagle’s medium (DMEM), fetal bovine serum (FBS), equine serum, neurobasal medium, B27 supplements, 0.25% trypsin–EDTA, and Hanks’ balanced salt solution for cell culture were from Invitrogen (Carlsbad, CA, USA). Poly-D-lysine, L-glutamine, tetraethylammonium chloride (TEA-Cl), tetrodotoxin (TTX), bicuculline, 6,7-dinitroquinoxaline-2,3-dione (DNQX), aminophosphonobutyrate (APV), 4-aminopyridine (4-AP), ethylene glycol-bis(2-aminoethylether)-tetraacetic acid (EGTA), adenosine triphosphate disodium salt (Na2ATP), cadmium chloride (CdCl2), and cesium chloride (CsCl) were from Sigma (St. Louis, MO, USA). DS (powder, reference substance for content determination, HPLC ≥98%; molecular weight: 416.5) was from the China National Institute for the Control of Pharmaceutical and Biological Products (Beijing, China) and was dissolved in DMSO. Other reagents were purchased from domestic reagent companies.
Cell culture Hippocampal neuron cultures were prepared as previously described[15]. Briefly, hippocampal tissues from 17–19 d old fetal rats were dissected and treated with 0.15% trypsin–EDTA at 37 °C for 15–20 min. Trypsin was then removed with a Pasteur pipette. The single cell suspension was diluted to a density of 120 000 cells/mL in high glucose DMEM containing 5% FBS, 5% equine serum, and 0.5 mmol/L L-glutamine, and was plated in 35-mm, glass bottom dishes for subsequent microscopy imaging or in 35-mm, ordinary culture dishes for subsequent patch-clamp experiments. All dishes were pretreated with poly-D-lysine (20 mg/L in deionized water) overnight. The cultures were incubated at 37 °C in a humidified incubator with 5% CO2. After approximately 24 h, the medium was replaced by serum-free neurobasal medium containing B27 supplement and 0.5 mmol/L L-glutamine to inhibit the growth of glia cells. Every 3-4 d, half of the media was replaced. After plating, the cultures were then used for experiments 9–11 d in vitro (DIV).
Ca2+ imaging The hippocampal cells were loaded with 6 μmol/L Fluo-4-AM (Invitrogen, USA) in Krebs–Ringer’s saline (in mmol/L: 150 NaCl, 5 KCl, 2 CaCl2, 1 MgCl2, 10 glucose, and 10 HEPES, pH 7.4)[6] at 37 °C for 35 min, followed by 3 washes and a 15 min incubation for de-esterification of Fluo-4-AM before imaging. Cells grown on the 35 mm, glass bottom dishes were directly imaged on a Nikon inverted microscope (TE300; Tokyo, Japan) using a 40× numerical aperture 1.30 oil immersion Plan Fluor objective. A Lambda DG-4 high-speed wavelength switcher (Sutter Instruments, Novato, CA, USA) was used for the Fluo-4 excitation at 480 nm, and a cooled CCD camera (CoolSnap FX; Roper Scientific, Princeton, NJ, USA) was used for image acquisition. MetaFluor imaging software (Universal Imaging, Downingtown, PA, USA) was used for hardware control, image acquisition, and image analysis. Using a heater positioned near the microscope stage, the hippocampal cells were maintained at 25-30 °C during imaging. In a typical signal-recording process, the time-lapse recording of Ca2+ signals in the hippocampal neurons was performed for 2 min (as the control period) before and 15 min after the application of different chemicals; the sampling rate was 1 frame every 2 s with an exposure time of 80 ms and CCD binning of 4×4 (Figure 2A, 2B).
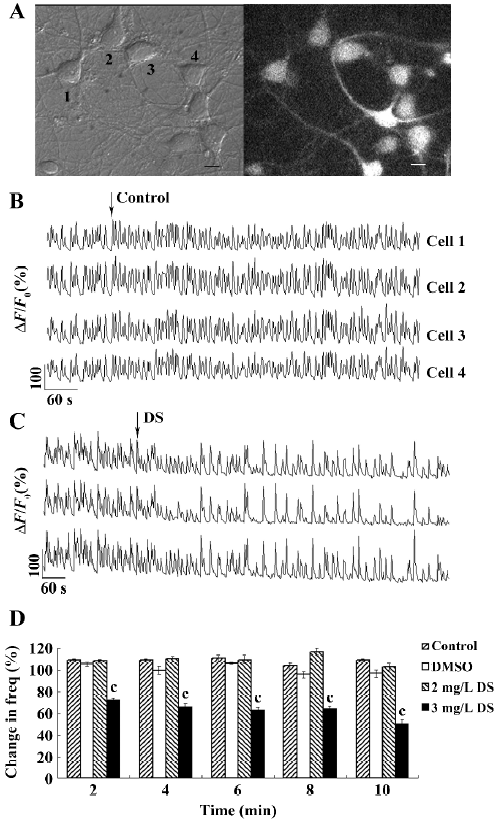
Quantitative analysis of frequency of synchronized Ca2+ spikes and spontaneous postsynaptic currents The quantitative measurements of [Ca2+]i were obtained by getting the average Fluo-4 fluorescence intensity of a 3×3 pixel2 analysis box placed at the centre of the cell body; the intensity values were then subtracted to the average background intensity measured in cell-free region. The changes of [Ca2+]i in each cell were represented by the changes of relative Fluo-4 fluorescence (ΔF/F0), where F0 was the baseline intensity. Ca2+ spikes were defined as the rapid elevation of ΔF/F0 ≥20%. Under our imaging settings, fields of 4-8 neurons were typically recorded and subsequently analyzed. Data from at least 3 dishes from different batches of cultures were pooled together and analyzed for significant statistical differences. We calculated the frequency of both the Ca2+ spikes and the spontaneous currents by counting the number of spikes and firings over the 2 min period of the recordings at a defined time point. The frequency values after the drug application were normalized to the control frequency value and expressed as percentages; a value of 100% indicated no change. Data were analyzed for significant statistical differences using paired Student’s t-test. The compiled data were expressed and graphed as mean±SEM, with n denoting the number of neurons studied. The SigmaPlot software (SPSS, Chicago, IL, USA) was used to fit the concentration–response data to the equation: Y=1/(1+[IC50/C]n), where C is the concentration of drug, Y the inhibition percentage, and n the Hill coefficient.
Bath application of different factors To examine the effects of DS on spontaneous calcium oscillation, the stock solution was diluted at least 1000 times to a 2× working concentration with Krebs–Ringer’s solution just before application. The dilution was applied to the cells to achieve the desired final concentration through 1:1 dilution (v/v). Specifically, we first recorded Ca2+ spikes for 2 min as the control period in 1 mL Krebs–Ringer’s solution, then added 1 mL of the 2×solutions, and recorded for another 15 min. For the control, we performed the same procedure and applied 1 mL Krebs–Ringer’s solution or 1 μL DMSO diluted in 1 mL Krebs–Ringer’s solution.
Electrophysiology Before the experiments, the culture dishes were rinsed twice and perfused with extracellular solution. For calcium currents recording, the extracellular solution contained the following (in mmol/L): 50 NaCl, 5 BaCl2, 90 TEA-Cl, 10 HEPES, 10 glucose, 5 4-AP, and 0.001 TTX, pH 7.3. For spontaneous excitatory postsynaptic currents (sEPSC), it contained the following (in mmol/L): 140 NaCl, 5 KCl, 1 MgCl2, 0.5 CaCl2, 10 glucose, 10 HEPES, and 0.05 bicuculline, pH 7.4. For spontaneous inhibitory postsynaptic currents, the bicuculline was replaced with 0.02 mmol/L DNQX and 0.05 APV. For potassium currents, the bicuculline was replaced with 0.001 mmol/L TTX and 0.4 mmol/L CdCl2 to block Na+ channel and Ca2+ and Ca2+-activated K+ channels, respectively. For sodium currents, the bicuculline was replaced with 5 mmol/L 4-AP. The intracellular pipette solution used for spontaneous postsynaptic currents and K+ current recordings contained the following (in mmol/L): 140 KCl, 10 HEPES, 10 EGTA, 2 MgCl2, 2 Na2ATP, and 1 CaCl2, pH 7.3. For Na+ and Ca2+ currents, it contained the following (in mmol/L): 140 CsCl, 10 HEPES, 10 EGTA, 2 MgCl2, 2 Na2ATP, and 1 CaCl2, pH 7.3. The whole-cell patch clamp experiments were performed at room temperature (22±2 °C) with the Axopatch-200B amplifier (Axon Instruments, Foster City, CA, USA). The patch electrodes (Fisherbrand, Pittsburgh, UK) were pulled on a PP-83 micropipette puller (Narishige, Tokyo, Japan). The typical resistance of glass electrodes was 4-7 MΩ when filled with the intracellular pipette solution. The signals were recorded with pClamp9 software (Axon Instruments, USA) and were analyzed with clampfit9 software (Axon Instruments, USA). All data were analyzed for significant statistical differences using paired Student’s t-test. The data were expressed as the mean±SEM.
Results
DS decreased the frequency of synchronized Ca2+ spikes To examine the effects of DS on synchronized Ca2+ spikes, we bath-applied DS to hippocampal cultures and recorded Ca2+ imaging before and after the application. We found that 2 mg/L (equal to 4.8 μmol/L) DS had no effect on the frequency (Figure 2D) or the amplitude of Ca2+ spikes (data not shown). However, 3 mg/L DS (equal to 7.2 μmol/L) induced a remarkable decrease in the frequency of Ca2+ spikes immediately after the application, but the amplitude of the Ca2+ spikes was not significantly affected (Figure 2C). After 1 min of DS application, the Ca2+ spike frequency was decreased to 72%±2% from that of the control period, and to 50%±4% at 9 min after application (Figure 2D). The bath application of the control saline buffer and the diluted DMSO did not affect the frequency of the Ca2+ spikes, indicating that no artifact was produced by the bath application method or by DMSO (Figure 2D). DS inhibited synchronized calcium oscillation in a dose-dependent manner (Figure 3). The concentration–response curve can be fitted well with a logistic equation. The IC50 value for the inhibitory effect of DS was calculated to be 3.8 mg/L.
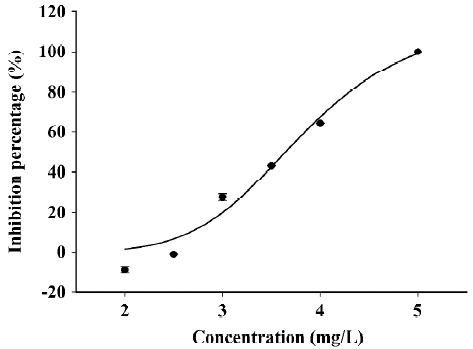
DS repressed voltage-gated sodium channel currents but had no effect on voltage-gated potassium channel currents It is reported that the spontaneous calcium spikes were the direct result of membrane depolarization from action potentials among synaptically-connected neurons[9], so we first examined the effect of DS on voltage-gated sodium and potassium channel currents. Electrophysiological experiments were performed on healthy, typical pyramidal hippocampal neurons using the whole-cell patch-clamp technique. Specifically, the cell was clamped at assumptive resting potential (–70 mV), and step depolarized to +60 mV in 10 mV increment. Since DS significantly decreased the calcium oscillation frequency after 1 min of application, we recorded currents every 2 min after DS application. Data showed that from 4 min after DS (3 mg/L) application, the voltage-gated sodium current amplitude was significantly decreased when membrane potential was depolarized from –40 mV to +60 mV (Figure 4A,4C). However, in the same course of time, the voltage-gated potassium current amplitude was not affected (Figure 4B, 4D).
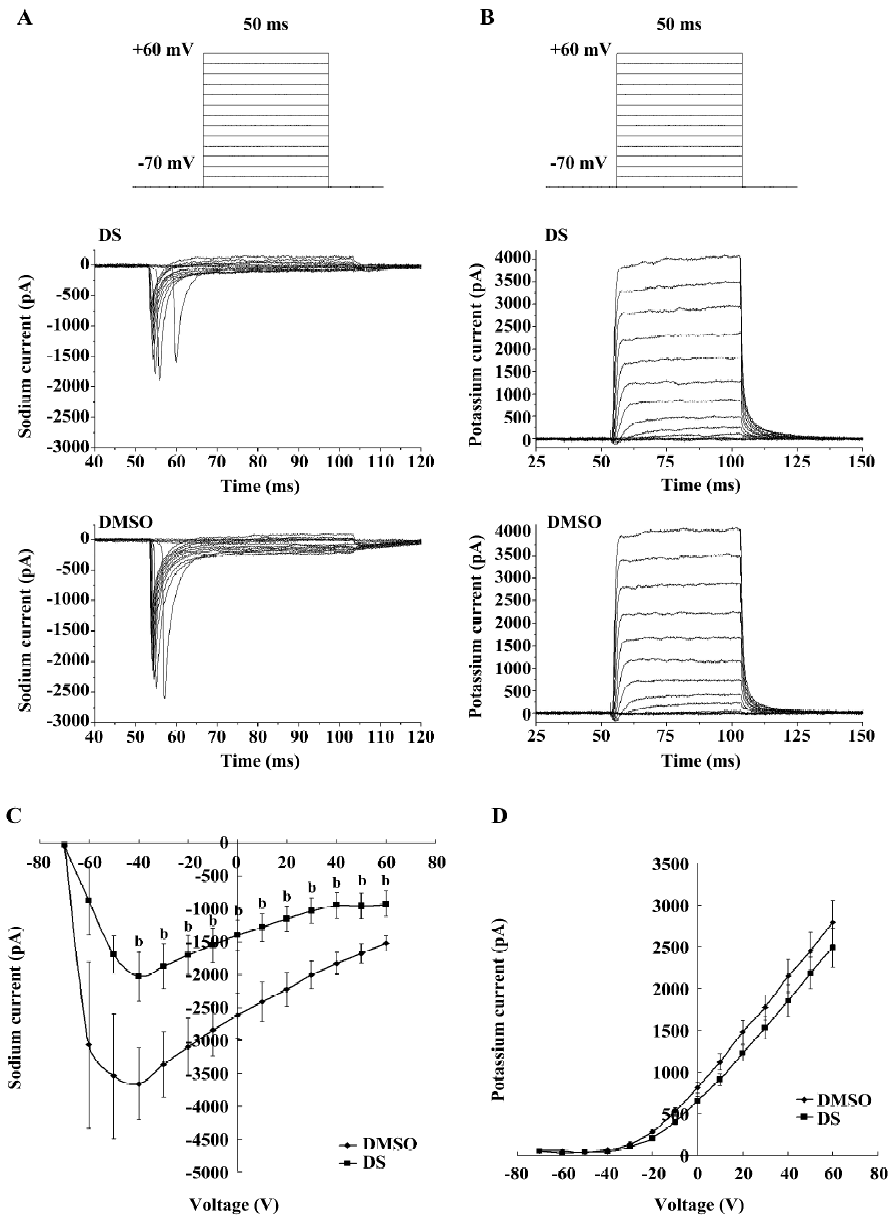
DS inhibited high voltage-activated calcium currents Since the influx of extracellular calcium shapes the synchronized oscillations, and the high voltage-activated (HVA) calcium channels are largely responsible for the regulation of calcium entry[16], we investigated the effect of DS on HVA calcium channels. The protocol was the same as that used in the recording of sodium and potassium currents. The cell was clamped at assumptive resting potential (–70 mV), and step depolarized to +60 mV in 10 mV increments. Ba2+ was used as the charge carrier in the extracellular solution to avoid Ca2+-induced inactivation. We also recorded the HVA calcium current every 2 min after DS application. The statistics indicated that the current amplitude was significantly decreased 6 min after DS (final 3 mg/L) application when membrane potential was depolarized from –10 mV to +60 mV (Figure 5).
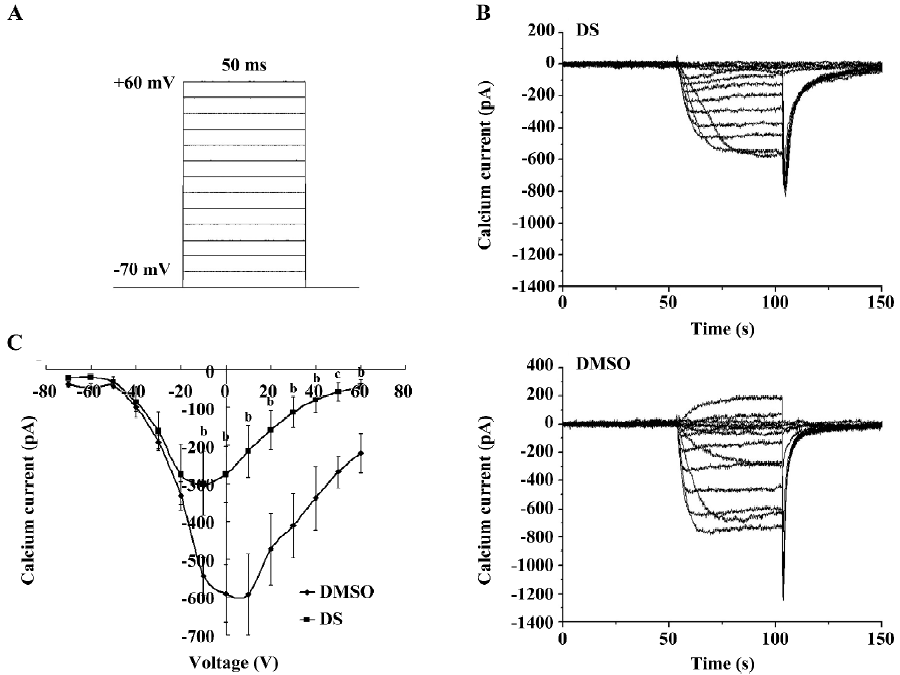
DS inhibited spontaneous inhibitory postsynaptic currents but not spontaneous excitatory postsynaptic currents The HVA calcium channels require strong membrane depolarization for gating and are largely responsible for neurotransmitter release from presynaptic nerve terminals. Because of this, we then investigated whether DS had influence on spontaneous synaptic transmission. At the time point that HAV calcium currents were inhibited, DS had no influence on the frequency and amplitude of sEPSC (Figure 6A,6C), but had a remarkable inhibitory effect on sIPSC (Figure 6B). The frequency of sIPSC was decreased to 60%±3% (mean±SEM) after 2 min of drug application, and to 42%±1% (mean±SEM) after 4 min of drug application (Figure 6D).
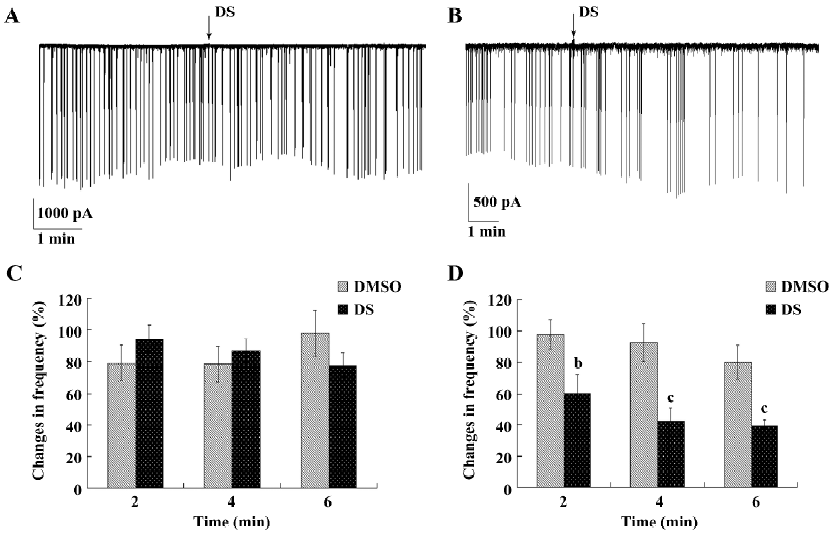
Discussion
In this study, we found that an appropriate concentration of DS effectively decreased the frequency of intracellular Ca2+ oscillation in cultured rat hippocampal neurons and remarkably inhibited voltage-gated sodium and HVA Ca2+ channel current amplitude. The whole-cell inward currents of sodium and calcium were blocked by 36% and 48%, respectively. The spontaneous neurotransmitter release in the neuronal network was repressed in terms of decreased sIPSC immediately after the application of DS.
It has been shown that primary hippocampal cultures mature after ~1 week and form functional synaptic connections to exhibit spontaneous synaptic transmission [17]. Spontaneous Ca2+ spikes have been previously reported to result from action potential firing among synaptically-connected neurons[6] and have also been reported to be blocked by TTX, blocker of neuronal action potentials[9]. The cascade activation of voltage-gated sodium channels initiate action potential firing and propagating. The inhibition of the influx of sodium cation consequently prevents generating the upstroke of action potentials. DS effectively inhibiting voltage-gated sodium is suggested to be one of the main reasons for its inhibitory effect on intracellular Ca2+ oscillation.
In the central nervous system, calcium channels are broadly grouped into HVA and low voltage-activated families. HVA channels require strong membrane depolarization for gating, and it has previously been reported that HVA calcium channels allow calcium from entering from the extracellular medium and from sustaining the long depolarization[6]. The data showed that DS remarkably decreased the current amplitude of the HVA calcium channels (Figure 5), indicating that the inhibitory effect of DS on extracellular calcium influxes contributed to the decrease of intracellular calcium oscillation.
Considering that the modulatory action of DS on synaptic transmission may involve direct action on neurotransmitter release in addition to the effects on ion channels, we further examined whether DS modulated spontaneous neurotransmitter release and recorded sEPSC and sIPSC separately. The sEPSC were composed of an equal mixture of TTX-insensitive miniature EPSC and EPSC that appeared to result from spontaneous action potentials. The detection of all sEPSC was glutamate-mediated synaptic events. DS had no effect on the frequency and amplitude of sEPSC, indicating that it may not be involved in presynaptic glutamate release[18]. Interestingly, DS remarkably decreased the frequency of sIPSC to 42%±8%, with no influence on the amplitude. One possible explanation for the different effect of DS on sEPSC and sIPSC could be a selective interaction between DS and the specific synaptic γ-aminobutyric acid (GABA) receptors. DS decreased the frequency of sIPSC, but had no effect on their amplitude, which indicated its selective action on GABAergic neurons and its inhibitory effect on the presynaptic release of GABA. It has been previously reported that voltage-gated calcium channels were involved in mediating GABAergic synaptic transmission[19]. We hypothesized that the inhibition of DS calcium influx underlies the inhibitory effect of DS on GABA release. However, this needs further investigation.
Interestingly, the inhibition of voltage-gated sodium and calcium currents indicated that DS downregulating neural excitability, in contrast to its inhibitory effect on sIPSC, which indicating the upregulation of neural excitability. It was suggested that the action of DS on neural network excitability would be bidirectional. Under normal physiological conditions, DS had no effect on the excitability of neural networks, yet in the case of pathological events, when neural networks were in perturbations, DS might play important roles to keep neural networks in homeostasis.
Even though Schisandra chinensis has been used for the treatment of several neurological disorders, only limited scientific evidence on its traditional use and mechanism of action has been reported. We demonstrated that DS decreased spontaneous and synchronous intracellular calcium oscillations in cultured hippocampal neural networks, resulting from the inhibitory effect of DS on voltage-gated sodium and HVA calcium channel currents. Spontaneous neurotransmitter release in the neuronal network was also repressed in terms of decreased sIPSC after the application of DS. These results provide further insights into the mechanisms involved in DS action on the central nervous system.
References
- Kashiwadani H, Sasaki YF, Uchida N, Mori K. Synchronized oscillatory discharges of mitral/tufted cells with different molecular receptive ranges in the rabbit olfactory bulb. J Neurophysiol 1999;82:1786-92.
- Buzsaki G, Draguhn A. Neuronal oscillations in cortical networks. Science 2004;304:1926-9.
- Shatz CJ. Impulse activity and the patterning of connections during CNS development. Neuron 1990;5:745-56.
- Salinas E, Sejnowski TJ. Correlated neuronal activity and the flow of neural information. Nat Rev Neurosci 2001;2:539-50.
- Traub RD, Jefferys JG. Are there unifying principles underlying the generation of epileptic afterdischarges in vitro? Prog Brain Res 1994;102:383-94.
- Bacci A, Verderio C, Pravettoni E, Matteoli M. Synaptic and intrinsic mechanisms shape synchronous oscillations in hippocampal neurons in culture. Eur J Neurosci 1999;11:389-97.
- Matteoli M, Verderio C, Krawzeski K, Mundigl O, Coco S, Fumagalli G, et al. Mechanisms of synaptogenesis in hippocampal neurons in primary culture. J Physiol Paris 1995;89:51-5.
- Arimura N, Kaibuchi K. Neuronal polarity: from extracellular signals to intracellular mechanisms. Nat Rev Neurosci 2007;8:194-205.
- Liu Z, Geng L, Li R, He X, Zheng JQ, Xie Z. Frequency modulation of synchronized Ca2+ spikes in cultured hippocampal networks through G-protein-coupled receptors. J Neurosci 2003;23:4156-63.
- Chiu PY, Tang MH, Mak DH, Poon MK, Ko KM. Hepatoprotective mechanism of schisandrin B: role of mitochondrial glutathione antioxidant status and heat shock proteins. Free Radic Biol Med 2003;35:368-80.
- Li L, Lu Q, Shen Y, Hu X. Schisandrin B enhances doxorubicin-induced apoptosis of cancer cells but not normal cells. Biochem Pharmacol 2006;71:584-95.
- Fujihashi T, Hara H, Sakata T, Mori K, Higuchi H, Tanaka A. Anti-human immunodeficiency virus (HIV) activities of halogenated gomisin J derivatives, new nonnucleoside inhibitors of HIV type 1 reverse transcriptase. Antimicrob Agents Chemother 1995;39:2000-7.
- Kim SR, Lee MK, Koo KA, Kim SH, Sung SH, Lee NG, et al. Dibenzocyclooctadiene lignans from Schisandra chinensis protect primary cultures of rat cortical cells from glutamate-induced toxicity. J Neurosci Res 2004;76:397-405.
- Nakajima K, Taguchi H, Ikeya Y, Endo T, Yosioka I. Constituents of Schizandra chinensis Baill. XIII. Quantitative analysis of lignans in the fruits of Schizandra chinensis Baill. by high performance liquid chromatography. Yakugaku Zasshi 1983;103:743-9.
- Banker GA, Cowan WM. Rat hippocampal neurons in dispersed cell culture. Brain Res 1977;126:397-42.
- Turner TJ. Calcium channels coupled to glutamate release. Prog Brain Res 1998;116:3-14.
- Verderio C. Synaptogenesis in hippocampal cultures. Cell Mol Life Sci 1999;55:1448-62.
- Cormier RJ, Kelly PT. Glutamate-induced long-term potentiation enhances spontaneous EPSC amplitude but not frequency. J Neurophysiol 1996;75:1909-18.
- Xiao C, Gu Y, Zhou CY, Wang L, Zhang MM, Ruan DY. Pb2+ impairs GABAergic synaptic transmission in rat hippocampal slices: A possible involvement of presynaptic calcium channels. Brain Res 2006;1088:93-100.