Two isoforms of cyclooxygenase contribute to augmented endothelium-dependent contractions in femoral arteries of 1-year-old rats1
Introduction
The endothelial cells lining the interior surface of the blood vessels modulate the tone of the underlying smooth muscle by releasing endothelium-derived relaxing (EDRF) and endothelium-derived contracting (EDCF) factors. EDCF, a hallmark of endothelial dysfunction, is a product of cyclooxygenase (COX) and activates thromboxane-prostanoid receptors of the vascular smooth muscle[1−8]. Endothelium-dependent contractions are augmented by aging[9−10], hypertension[1,3,4,9,11], vasospasm[12], high glucose[13], and diabetes[8,14].
Cardiovascular diseases augment in frequency with aging even in the absence of established risk factors, suggesting that aging per se alters vascular function[15]. Endothelium-dependent relaxations are impaired with advancing age in different species, including humans[10,16−18]. This could be due to a reduced nitric oxide bioavailability, decreased endothelium-dependent hyperpolarizations, or augmented endothelium-dependent contractions.
The increased production of oxygen-derived free radicals is an important characteristic of aging[15,19,20]. Reactive oxygen-derived free radicals not only reduce endothelium-dependent relaxations by scavenging nitric oxide[21], but also play a crucial role in endothelium-dependent contractions[3,14,22,23]. Thus, superoxide anion has been proposed as an endothelium-derived contracting factor in the canine basilar arteries[22] and hydroxyl radicals in the aorta of spontaneously hypertensive rats[3] or the femoral arteries of diabetic rats[14]. In addition to their mediator role, oxygen-derived free radicals facilitate endothelium-dependent contractions in the aorta of spontaneously hypertensive rats and rabbits[3,11,23,24] and the femoral arteries of diabetic rats[14].
COX metabolizes arachidonic acid, leading to the production of prostaglandins and thromboxane A2. In addition, COX can produce reactive oxygen species (ROS)[14,19,23,25]. COX-1, the constitutive isoform of the enzyme, plays a key role in the regulation of the physiological functions mediated by prostanoids in most cells. It can also be induced or upregulated by shear stress[26], and its upregulation is responsible for the release of endothelium-derived contracting factor in the aorta of spontaneously hypertensive rats[27] and in the femoral arteries of rats with streptozotocin-induced type I diabetes[8]. COX-2, the inducible isoform, can be induced by reactive oxidative species and contributes to endothelial dysfunction with aging[17,28,29,30].
These findings prompted the hypothesis that reactive oxygen-derived free radicals are involved in endothelium-dependent contractions in aging as they are in disease. Therefore, the present experiments were designed to study how aging affects endothelium-dependent contractions of the rat femoral arteries. Special attention was paid to the contribution of oxidative stress and that of the 2 isoforms of COX.
Materials and methods
Experimental animals All experiments were performed on the isolated femoral arteries of Sprague-Dawley rats. Two groups of rats of different ages (20 weeks and 1 year) were housed in the Laboratory Animal Unit of the University of Hong Kong (Hong Kong), fed regular chow, and given free access to water. On the day of the experiments, they were anesthetized with intraperitoneal pentobarbitone sodium (70 mg/kg), anticoagulated with heparin (0.5 U/kg), and exsanguinated. The Institutional Animal Care Committee approved all procedures and protocols.
Tissue preparation The femoral arteries were dissected, excised, and placed into ice-cold modified Krebs-Ringer solution of the following composition (mmol/L): NaCl 118, KCl 4.7, CaCl2 2.5, MgSO4 1.2, NaHCO3 25.0, KH2PO4 1.18, calcium disodium EDTA 0.026, and glucose 11.1 (control solution). The blood vessels were cut into rings (1.5−2 mm in length). When the arterial rings without endothelium were needed, the arteries were perfused with 1 mL saponin solution (1 mg/mL, diluted with Krebs–Ringer solution)[31] before the rings were cut. The rings were suspended in organ chambers containing control solution (37 °C) aerated with 95% O2 and 5% of CO2. They were connected to a force transducer (Powerlab Model ML785 and ML119, ADInstruments, Inc., Colorado Spring, CO, USA). Changes in isometric tension were recorded. The rings were stretched progressively to their optimal resting tension (1.0 g; determined in preliminary experiments) and were allowed to equilibrate for 90 min before experimentation. Changes in tension were expressed as a percentage of the reference contraction to 60 mmol/L KCl (isotonic solution), obtained at the beginning of the experiment.
The incubation time with drugs was 30 min, and the concentration-response curves were obtained in a cumulative way. To study the endothelium-dependent relaxations, the preparations were exposed to phenylephrine (0.1–1 µmol/L; in order to obtain 50%−70% of the response to KCl). Sodium nitroprusside (10 µmol/L) was added at the end of the experiments, and the relaxations were expressed as a percentage of the maximal relaxation induced by the nitrovasodilator. To study the endothelium-dependent contractions, all experiments were performed in the presence of Nω-nitro-L-arginine methyl ester hydrochloride (L-NAME; 0.3 mmol/L)[4,32,33].
Western blotting The arteries were dissected and cleaned of connective tissue. To remove the remaining blood cells, the arteries were rinsed 3 times in Krebs-Ringer solution. The blood vessels were homogenized at 4 ºC in lysis buffer (10 mmol/L Tris-HCl, 150 mmol/L NaCl, 1 mmol/L EDTA, 25 mmol/L sodium pyrophosphate, 1 mmol/L β-glyco-phosphate, 1 mmol/L sodium orthovanadate, 2.1 µmol/L leupeptin, 1 mg/mL aprotinin, 1 mmol/L phenyl-methylsulfonyl fluoride, and 1% Triton X-100) and incubated on ice for 10 min. The samples were then centrifuged at 3000×g for 10 min at 4 ºC and the supernatant was collected. Protein concentrations were determined using the Bradford assay. The lysates were used immediately or stored at –80 ºC. In all the immunoblot experiments, equal amounts of protein were loaded in each lane of the SDS–PAGE gels. The molecular masses of the immunoreactive bands were determined by loading a biotinylated molecular mass standard (Bio-Rad Laboratories, Hercules, CA, USA). After electrophoresis, the proteins were transferred to nitrocellulose membranes (Bio-Rad Laboratories, USA) and blocked with 5% non-fat, dry milk in Tris buffered saline (TBS; 20 mmol/L Tris-HCl, and 140 mmol/L NaCl, pH 7.4) for 1 h. The membranes were then incubated with the suggested dilution of corresponding primary antibodies (COX-1, 1:300; COX-2, 1:500) overnight at room temperature, followed by 3×10 min washes in 0.1% Tween 20-TBS (T-TBS). The blots were incubated with secondary antibodies at 1:1000 dilutions for 2 h at room temperature, and then washed 3 times for 10 min in T-TBS. The membranes were then incubated with an enhanced chemiluminescence (ECL) reagent (Amersham Biosciences, Buck-inghamshire, UK) for 1 min and exposed to X-ray films (Fuji Super RX medical X-ray films; Fuji Photo Film, Dusseldorf, Germany) for the recommended optimal times, depending on the signal strength. The software for the electrophoresis analysis (Multi-analyst, Bio-Rad Laboratories) was used for the densitometric measurement of the immunoreactive bands. The presence of the protein was normalized to β-actin. All protein presences were expressed as the percentage of the control.
Fluorescence studies ROS were measured with 2',7'-dichlorodihydrofluorescein diacetate (DCF). Non-ionized DCF is permeable of membrane and therefore diffuses readily into cells. The fluorescence experiments were performed in a dark room. The rings of the femoral arteries were loaded with DCF solution (10 µmol/L) for 15 min followed by incubation with the tested agents for 30 min. After rinsing 3 times in control solution, the rings were opened longitudinally and placed with the endothelial layer down on a microscopic stage. ROS were measured using a confocal laser-scanning microscope. The intensity of the production was recorded after excitation at 488 nm and emission at 510 nm using the appropriated software (Laser Sharp 2000, Bio-Rad Cell Science Division Hemel Hempstead, UK). The production of ROS measured from 9 randomly-related fields were averaged in the endothelium and the vascular smooth muscle of each preparation. To correct for non-specific changes in fluorescence, the background signal was subtracted from the measurements and only the changes induced by A23187 are reported and discussed[14].
Enzymatic activities The arteries were dissected and cleaned of connective tissue. The preparations were homogenized at 4 ºC in lysis buffer (50 mmol/L potassium phosphate containing 1 mmol/L EDTA, pH 7.0). The samples were centrifuged at 10 000×g for 10 min at 4 ºC, and the supernatant was collected. Protein concentrations were determined using the Bradford assay. The lysates were used immediately or stored at –80 ºC. The level of glutathione and catalase activity was measured following the instructions of the manufacturers.
Reagents Acetylcholine, anti-β-actin monoclonal antibody, calcimycin (A23187) DCF, indomethacin, L-NAME, saponin, and sodium nitroprusside were purchased from Sigma (St Louis, MO, USA). NS-398 (N[-2-(cyclohexyloxy)-4-nitrophenyl]-methanesulphonamide), valeryl salicylate (2-[(1-oxopenytyl)oxy]-benzoic acid), glutathione detection kits, catalase detection kits, COX-1 monoclonal antibodies, and COX-2 polyclonal antibodies were purchased from Cayman (Ann Arbor, MI, USA). Heparin was purchased from LEO Pharma (Ballerup, Denmark). Secondary antibodies [ECL antirabbit immunoglobulin G (IgG) and ECL antimouse IgG] were obtained from Amersham Biosciences (Bucking-hamshire, UK). Terutroban, S18886, (3-[(6-amino-(4-chlorobenzensulphonyl)-2-methyl-5,6,7,8-tetrahydro-napht]-1-yl) propionic acid) was a kind gift of the Institut de Recherche Servier (Paris, France). Drug concentrations are given as final molar concentrations in the bath solution.
Data analysis Data are presented as mean±SEM; n refers to the number of rats. The statistical analysis was done by one-way ANOVA followed by post-hoc comparison using the Bonferroni test, or by two-way ANOVA, as appropriate (Prism version 3a, GraphPad Software, San Diego, CA, USA). Differences were considered to be statistically significant when P was less than 0.05.
Results
General condition At the beginning of experiments, the body weight was comparable in both groups. With aging, the rats gradually gained body weight and the 1-year-old rats were significantly heavier than the younger ones (20 weeks 684.5±23.6 g, 1 year 840.0±21.6 g, P<0.05).
Endothelium-dependent relaxations Acetylcholine induced a concentration-dependent relaxation (Figure 1A,1B). The acetylcholine-induced relaxation was slightly, but significantly reduced in the arteries from the 1-year-old rats. Indomethacin (an inhibitor of COX, 5 µmol/L) prevented the reduced response in the arteries from the 1-year-old group (Figure 1B).
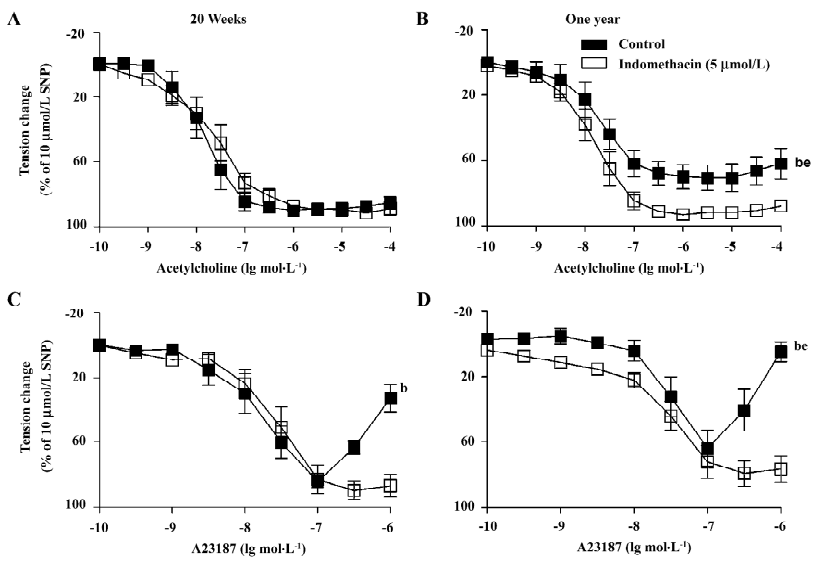
The calcium ionophore A23187 induced a concentration-dependent relaxation at lower concentrations (0.1 nmol/L–0.1 µmol/L) and a secondary increase in tension at higher (0.3–1 µmol/L) concentrations (Figure 1C,1D). In the arteries from the 1-year-old rats, A23187 induced a comparable relaxation, but an enhanced secondary contraction. The secondary contraction was inhibited by indomethacin (Figure 1D).
Endothelium-dependent contractions In the presence of L-NAME (an inhibitor of nitric oxide synthase, 0.3 mmol/L), quiescent rings with endothelium of 20-week and 1-year-old rats did not contract when exposed to increased concentrations of acetylcholine (data not shown). Under the same conditions, A23187 induced concentration-dependent contractions in rings with, but not in those without endothelium. The contractions in rings with endothelium were significantly greater in the arteries from the 1-year–old rats than that in those from the younger rats (Figure 2). The endothelium-dependent contractions were abolished by indomethacin and terutroban (a blocker of thromboxane-prostanoid receptor, 0.1 µmol/L; Figure 3A). Valeryl salicylate or NS-398 (preferential inhibitors of COX-1, 3 mmol/L and COX-2, 1 µmol/L, respectively) partially inhibited the contraction to A23187 in rings with endothelium (Figure 3B).
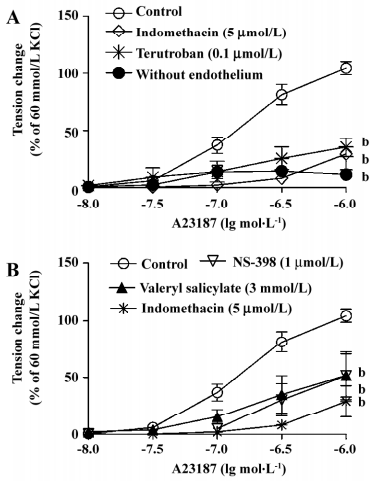
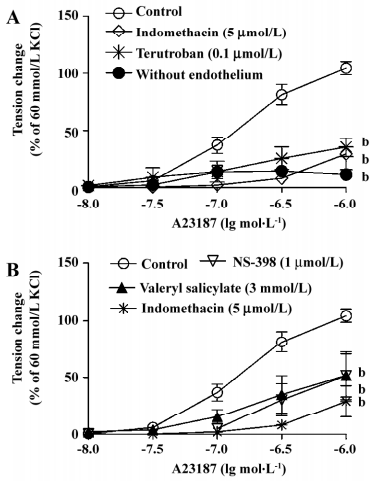
DCF fluorescence study In the presence of L-NAME (0.3 mmol/L), the calcium ionophore A23187 (0.3 µmol/L) increased the fluorescence intensity in the endothelium of the arteries of the 1-year–old rats, but not of those of the 20-week-old rats. This increase was reduced significantly by indomethacin (Figure 4, right), but not by terutroban (data not shown). A23187 did not significantly increase the fluorescence intensity in the underlying smooth muscle in either group (data not shown).
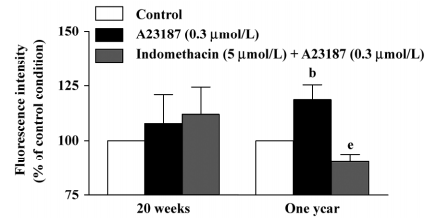
Western blotting The protein levels of COX-1 in the arteries with endothelium from the 1-year-old rats was significantly higher than that in preparations from the 20-week-old animals (Figure 5A,5B), but was comparable in the arteries without endothelium of the 2 groups(data not shown).
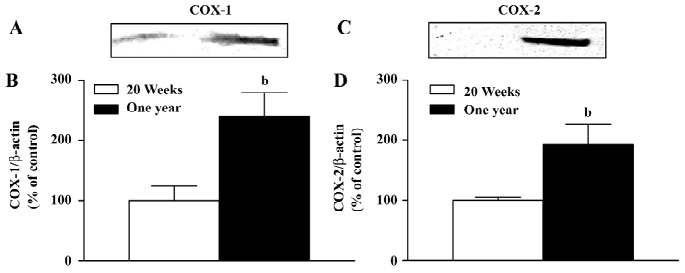
The protein level of COX-2 was increased in the arteries with endothelium from the 1-year-old rats (Figure 5C,5D), while it was comparable in the arteries without endothelium of the 2 groups (data not shown).
Catalase and glutathione The activity of catalase was reduced significantly in the femoral arteries with endothelium from the 1-year-old rats (Figure 6A).The global level of glutathione was not significantly different in the femoral arteries with endothelium in the 2 groups (Figure 6B).
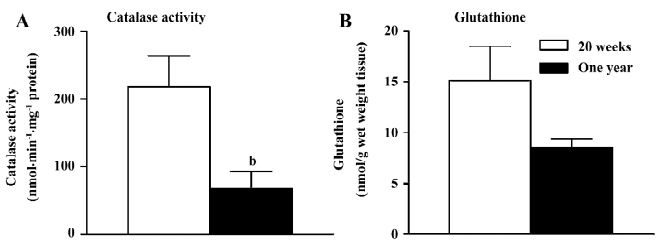
Discussion
The present experiments were designed to study the endothelial function in the process of aging. Endothelial dysfunction is attributed to either impaired endothelium-dependent relaxations, augmented endothelium-dependent contractions, or both[34]. The relaxation induced by the receptor-mediated agonist acetylcholine was slightly, but significantly reduced in the femoral arteries from the 1-year-old rats. Indomethacin, an inhibitor of COX, restored the blunted response, suggesting that an endothelium-dependent contracting factor derived from COX exists in the femoral arteries from 1-year-old rats. For the receptor-independent response to the calcium ionophore A23187[35], the concentration-dependent relaxation was comparable between the groups, but the secondary concentration-dependent contraction was significantly higher in the arteries from the 1-year-old rats than in that from the younger rats. The secondary contraction was also prevented by indomethacin, which is in agreement with the data of acetylcholine-induced relaxation. Thus, these results confirm that endothelial dysfunction with aging can be attributed to the occurrence of enhanced endothelium- and COX-dependent contractions[10,17,29].
To further study endothelium-dependent contractions in rat femoral arteries, all of the experiments were performed with quiescent preparations in the presence of L-NAME, an inhibitor of nitric oxide synthase, since nitric oxide exerts negative feedback on this response[4,32,33]. Endothelium-dependent contractions were obtained with A23187, but not acetylcholine. This heterogeneity in response is consistent with the findings in the same arteries from rats with streptozotocin-induced type I diabetes[8]. The difference in response to A23187 and acetylcholine could be explained by different increases in intracellular calcium in the endothelium evoked by these 2 agents[23]. Therefore, the calcium ionophore A23187 was used as a tool to investigate the endothelium-dependent contractions in further experiments. The endothelium-dependent response was augmented in older rats, confirming that aging enhances the production or the action of the endothelium-derived contracting factor[10]. The endothelium-dependent contractions observed in the present study must be due to the production of COX and the subsequent activation of thromboxane-prostanoid receptors on the vascular smooth muscle, since indomethacin and terutroban, a blocker of these receptors[36], completely prevented the response[1,8,37]. However in aging, unlike in the aorta of the spontaneously hypertensive rats[3] and the femoral arteries of diabetic rats[8], both COX-1 and COX-2 contribute to endothelium-dependent contractions, since at the concentrations used, either preferential inhibition of COX-1 (valeryl salicylate[3,38]) or COX-2 (NS-398[3,39]) reduced the response. The constitutive isoform COX-1 plays a prominent role in the augmented endothelium-dependent contraction in hypertension[3,27] and type I diabetes[8]. COX-2, the inducible isoform of COX, is present in macrophages and vascular smooth muscle in response to inflammatory stress, cytokines, and oxidative stress[42]. It is upregulated in the senescent endothelium[17,30] and by atherosclerosis[40–43]. The increased presence of both COX-1 and COX-2 observed in the present study with Western blotting supports an active contribution of both COX-1 and COX-2 in the occurrence of endothelium-dependent contractions with aging. The data of the present study concur with the results obtained in the aorta of 2-year-old Wister-Kyoto rats where both the constitutive and inducible isoforms of COX were involved in the blunted endothelium-dependent relaxations observed with aging[17,29].
The oxidative state was studied in the present study with fluorescence measurement under confocal microscopy and the measurement of catalase activity and glutathione levels. Both glutathione and catalase transform hydrogen peroxide to water[25]. Since the activity of catalase, but not the glutathione net level was significantly reduced in the arteries from the 1-year-old rats, the present data indicate that the reduced activity of catalase is a likely source of oxidative stress in the endothelium with aging as it is in the same artery from rats with streptozotocin-induced diabetes[14]. In the presence of L-NAME, the calcium ionophore A23187 increased the fluorescence of ROS. An increased production of ROS in response to A23187 during the inhibition of nitric oxide has been reported in the aorta of the spontaneously hypertensive rats and in the femoral arteries of diabetic rats[14,23]. Since indomethacin, but not terutroban, reduced the free radical release, these data are in line with the endothelium-dependent contraction observed in organ chambers and demonstrate that the ROS derived from COX inside the endothelium play an important role in the genesis of the endothelium-dependent contractions[3,14,22,23,44].
In conclusion, endothelium-dependent contractions are augmented in the femoral arteries of 1-year-old rats. Oxygen-derived free radicals take part in the occurrence of this response augmented by aging. Both the constitutive and inducible isoforms of COX contribute to this endothelial dysfunction.
References
- Lüscher TF, Vanhoutte PM. Endothelium-dependent contractions to acetylcholine in the aorta of the spontaneously hypertensive rat. Hypertension 1986;8:344-8.
- Auch-Schwelk W, Katusic ZS, Vanhoutte PM. Thromboxane A2 receptor antagonists inhibit endothelium-dependent contractions. Hypertension 1990;15:699-703.
- Yang D, Feletou M, Boulanger CM, Wu HF, Levens N, Zhang JN, et al. Oxygen-derived free radicals mediate endothelium-dependent contractions to acetylcholine in aortas from spontaneously hypertensive rats. Br J Pharmacol. 2002;136:104-10.
- Tang EH, Feletou M, Huang Y, Man RY, Vanhoutte PM. Acetylcholine and sodium nitroprusside cause long-term inhibition of EDCF-mediated contractions. Am J Physiol Heart Circ Physiol 2005;289:H2434-40.
- Vanhoutte PM, Feletou M, Taddei S. Endothelium-dependent contractions in hypertension. Br J Pharmacol 2005;144:449-58.
- Gluais P, Lonchampt M, Morrow JD, Vanhoutte PM, Feletou M. Acetylcholine-induced endothelium-dependent contractions in the SHR aorta: the Janus face of prostacyclin. Br J Pharmacol 2005;146:834-45.
- Gluais P, Paysant J, Badier-Commander C, Verbeuren T, Vanhoutte PM, Feletou M. In SHR aorta, calcium ionophore A-23187 releases prostacyclin and thromboxane A2 as endothelium-derived contracting factors. Am J Physiol Heart Circ Physiol 2006;291:H2255-64.
- Shi Y, Feletou M, Ku DD, Man RY, Vanhoutte PM. The calcium ionophore A23187 induces endothelium-dependent contractions in femoral arteries from rats with streptozotocin-induced diabetes. Br J Pharmacol 2007;150:624-32.
- Koga T, Takata Y, Kobayashi K, Takishita S, Yamashita Y, Fujishima M. Age and hypertension promote endothelium-dependent contractions to acetylcholine in the aorta of the rat. Hypertension 1989;14:542-8.
- Mombouli JV, Vanhoutte PM. Purinergic endothelium-dependent and -independent contractions in rat aorta. Hypertension 1993;22:577-83.
- Auch-Schwelk W, Katusic ZS, Vanhoutte PM. Contractions to oxygen-derived free radicals are augmented in aorta of the spontaneously hypertensive rat. Hypertension 1989;13:859-64.
- Dhein S, Salameh A, Klaus W. A new endothelium-dependent vasoconstricting factor (EDCF) in pig coronary artery. Eur Heart J 1989;10:82-5.
- Tesfamariam B, Brown ML, Deykin D, Cohen RA. Elevated glucose promotes generation of endothelium-derived vasoconstrictor prostanoids in rabbit aorta. J Clin Invest 1990;85:929-32.
- Shi Y, So KF, Man RY, Vanhoutte PM. Oxygen-derived free radicals mediate endothelium-dependent contractions in femoral arteries of rats with streptozotocin-induced diabetes. Br J Pharmacol 2007;152:1033-41.
- Van der Loo B, Labugger R, Skepper JN, Bachschmid M, Kilo J, Powell JM, et al. Enhanced peroxynitrite formation is associated with vascular aging. J Exp Med 2000;192:1731-44.
- Taddei S, Virdis A, Mattei P, Ghiadoni L, Fasolo CB, Sudano I, et al. Hypertension causes premature aging of endothelial function in humans. Hypertension 1997;29:736-43.
- Heymes C, Habib A, Yang D, Mathieu E, Marotte F, Samuel J, et al. Cyclo-oxygenase-1 and -2 contribution to endothelial dysfunction in ageing. Br J Pharmacol 2000;131:804-10.
- Matz RL, Schott C, Stoclet JC, Andriantsitohaina R. Age-related endothelial dysfunction with respect to nitric oxide, endothelium-derived hyperpolarizing factor and cyclooxygenase products. Physiol Res 2000;49:11-8.
- Finkel T, Holbrook NJ. Oxidants, oxidative stress and the biology of ageing. Nature 2000;408:239-47.
- Brandes RP, Kreuzer J. Vascular NADPH oxidases: molecular mechanisms of activation. Cardiovasc Res 2005;65:16-27.
- Rubanyi GM, Vanhoutte PM. Superoxide anions and hyperoxia inactivate endothelium-derived relaxing factor. Am J Physiol 1986;250:H822-827.
- Katusic ZS, Vanhoutte PM. Superoxide anion is an endothelium-derived contracting factor. Am J Physiol 1989;257:H33-37.
- Tang EH, Leung FP, Huang Y, Feletou M, So KF, Man RYK, et al. Calcium and reactive oxygen species increase in endothelial cells in response to releaser of endothelium-derived contracting factor. Br J Pharmacol 2007;151:15-23.
- Tesfamariam B, Cohen RA. Free radicals mediate endothelial cell dysfunction caused by elevated glucose. Am J Physiol 1992;263:H321-6.
- Wolin MS. Subcellular localization of Nox-containing oxidases provides unique insight into their role in vascular oxidant signaling. Arterioscler Thromb Vasc Biol 2004;24:625-7.
- Doroudi R, Gan LM, Selin Sjogren L, Jern S. Effects of shear stress on eicosanoid gene expression and metabolite production in vascular endothelium as studied in a novel biomechanical perfusion model. Biochem Biophys Res Commun 2000;269:257-64.
- Ge T, Hughes H, Junquero DC, Wu KK, Vanhoutte PM, Boulanger CM. Endothelium-dependent contractions are associated with both augmented expression of prostaglandin H synthase-1 and hypersensitivity to prostaglandin H2 in the SHR aorta. Circ Res 1995;76:1003-10.
- de Sotomayor MA, Pérez-Guerrero C, Herrrera MD, Jimenez L, Marín R, Marhuenda E, et al. Improvement of age-related endothelial dysfunction by simvastatin: effect on NO and COX pathways. Br J Pharmacol 2005;146:1130-8.
- Kang KB, Rajanayagam MA, van der Zypp A, Majewski H. A role for cyclooxygenase in aging-related changes of beta-adrenoceptor-mediated relaxation in rat aortas. Naunyn Schmiedebergs Arch Pharmacol 2007;375:273-81.
- Gendron ME, Thorin-Trescases N, Villeneuve L, Thorin E. Aging associated with mild dyslipidemia reveals that COX-2 preserves dilation despite endothelial dysfunction. Am J Physiol Heart Circ Physiol 2007;292:H451-8.
- Samata K, Kimura T, Satoh S, Watanabe H. Chemical removal of the endothelium by saponin in the isolated dog femoral artery. Eur J Pharmacol 1986;128:85-91.
- Auch-Schwelk W, Katusic ZS, Vanhoutte PM. Nitric oxide inactivates endothelium-derived contracting factor in the rat aorta. Hypertension 1992;19:442-5.
- Yang D, Gluais P, Zhang JN, Vanhoutte PM, Feletou M. Nitric oxide and inactivation of the endothelium-dependent contracting factor released by acetylcholine in spontaneously hypertensive rat. J Cardiovasc Pharmacol 2004;43:815-20.
- Feletou M, Vanhoutte PM. Endothelial dysfunction: a multifaceted disorder (The Wiggers Award Lecture). Am J Physiol Heart Circ Physiol 2006;291:H985-1002.
- Singer HA, Peach MJ. Calcium- and endothelial-mediated vascular smooth muscle relaxation in rabbit aorta. Hypertension 1982;4:19-25.
- Simonet S, Descombes JJ, Vallez MO, Dubuffet T, Lavielle G, Verbeuren TJ. S. 18886, a new thromboxane (TP)-receptor antagonist is the active isomer of S 18204 in all species, except in the guinea-pig. Adv Exp Med Biol 1997;433:173-6.
- Yang D, Félétou M, Levens N, Zhang JN, Vanhoutte PM. A diffusible substance(s) mediates endothelium-dependent contractions in the aorta of SHR. Hypertension 2003;41:143-8.
- Bhattacharyya DK, Lecomte M, Dunn J, Morgans DJ, Smith WL. Selective inhibition of prostaglandin endoperoxide synthase-1 (cyclooxygenase-1) by valerylsalicylic acid. Arch Biochem Biophys 1995;317:19-24.
- Futaki N, Takahashi S, Yokoyama M, Arai I, Higuchi S, Otomo S. NS-398, a new anti-inflammatory agent, selectively inhibits prostaglandin G/H synthase/cyclooxygenase (COX-2) activity in vitro. Prostaglandins 1994;47:55-9.
- Warner TD, Mitchell JA. Cyclooxygenases: new forms, new inhibitors, and lessons from the clinic. FASEB J 2004;18:790-804.
- Baker CS, Hall RJ, Evans TJ, Pomerance A, Maclouf J, Creminon C, et al. Cyclooxygenase-2 is widely expressed in atherosclerotic lesions affecting native and transplanted human coronary arteries and colocalizes with inducible nitric oxide synthase and nitrotyrosine particularly in macrophages. Arterioscler Thromb Vasc Biol 1999;19:646-55.
- Schonbeck U, Sukhova GK, Graber P, Coulter S, Libby P. Augmented expression of cyclooxygenase-2 in human atherosclerotic lesions. Am J Pathol 1999;155:1281-91.
- Belton O, Byrne D, Kearney D, Leahy A, Fitzgerald DJ. Cyclooxygenase-1 and -2-dependent prostacyclin formation in patients with atherosclerosis. Circulation 2000;102:840-5.
- Armstead WM. Cyclooxygenase-2-dependent superoxide generation contributes to age-dependent impairment of G protein-mediated cerebrovasodilation. Anesthesiology 2003;98:1378-83.