Plastins: versatile modulators of actin organization in (patho)physiological cellular processes
Introduction
The actin cytoskeleton of eukaryotic cells is a dynamic meshwork that is involved in many biological phenomena, such as cell motility, cell substrate adhesion, intracellular transport, endo-, and exocytosis, cytokinesis, and cell morphology. The overall organization of the actin cytoskeleton is controlled by a plethora of actin-binding proteins[1]. Plastins belong to a class of actin-bundling proteins, and they are conserved from lower eukaryotes to humans. In vertebrates, three different plastin isoforms are expressed in a cell-type-specific manner, and these isoforms display distinct properties. Here we review the discovery, biological properties and regulation of plastin isoforms, and, where appropriate, discuss their interest from a medical perspective.
Discovery
The first plastin isoform was discovered in 1979[2] in microvilli isolated from chicken intestinal brush border as a 68 kDa polypeptide involved in microfilament organization of the microvilli core bundle. This protein was named fimbrin because it was associated with surface structures such as membrane ruffles, microvilli, microspikes and focal adhesions in chicken embryo fibroblasts and cultured rat mammary cells[3]. Chicken fimbrin was characterized as a monomeric cytoske-letal protein able to bind and cross-link F-actin filaments[4,5], promoting the formation of rigid straight bundles in which all actin filaments have the same polarity.
Meanwhile, a 68 kDa protein was identified in transformed human fibroblasts (neoplastic cells, hence the name)[6,7]. This isoform, L-plastin, is also abundantly expressed in untrans-formed lymphocytes[8]. Molecular cloning of their corresponding cDNAs and amino acid sequence comparison revealed two highly related proteins with a predicted molecular weight of 64 kDa. These two human cell type-specific isoforms, L- and T-plastin (80% amino acid identity), are expressed in hematopoietic cells and in cells derived from solid tissue, respectively[9]. In 1990, it was observed that an amino-terminal sequence containing a potential calcium binding domain was missing in the sequences reported earlier[10,11]. A third human plastin isoform, called I-plastin, was discovered as a polypeptide that is specifically expressed in the small intestine, colon and kidney, and is 86% identical to the chicken fimbrin (plastin) isoform[12,13].
Plastin structure
Plastins have a modular structure consisting of 2 amino-terminal EF-hands, variably implicated in Ca2+-binding, and two tandem actin-binding domains, each divided into two calponin homology (CH) domains[14–16] (Figure 1). These CH domains are shared by both signaling and cytoskeletal proteins such as dystrophin, α-actinin, and spectrin, and a tandem CH domain is generally involved in actin-binding.
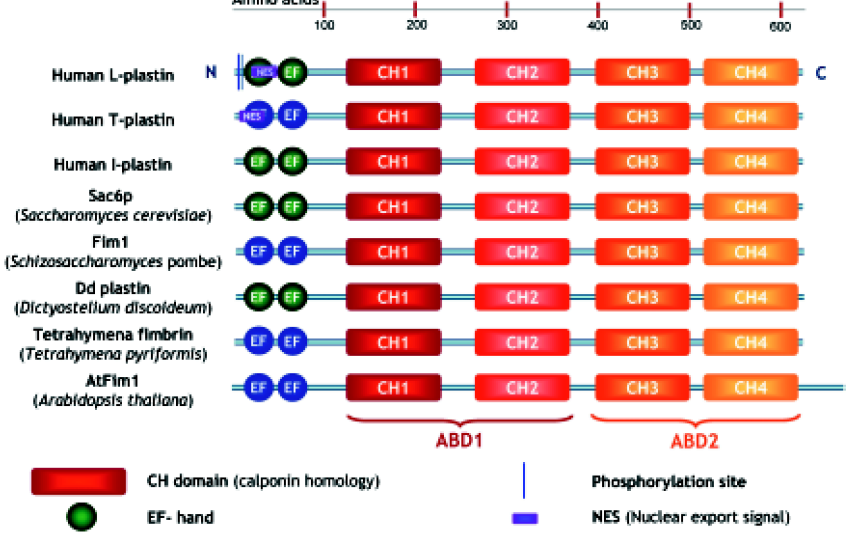
Each CH domain is composed of four α-helical segments, in which three form a loose bundle of helices, with the fourth α-helix perpendicular to the major bundle. These segments are connected by extended and variable loops and sometimes 2 additional short helices. The complete crystal structure of plastin has not yet been resolved, but the structure of the N-terminal actin-binding domain 1 (ABD1) of T-plastin and the complete cross-linking core of Arabidopsis thaliana plastin and of Schizosaccharomyces pombe plastin have been solved[17,18]. Additional methods, such as electron microscopy, image analysis and homology modeling, have led to a general model of the plastin structure and to a view of how this protein cross-links actin filaments.
The actin cross-linking core of plastin has a compact architecture[17], and the ABDs pack in such a way that the CH1 domain and the CH4 domain make contact, involving conserved residues on the molecular surface of the CH1–CH4 interface. Electron density in regions connecting the CH domains is poorly defined, indicating that these segments are highly dynamic. The potential structural plasticity of ABD1 by reorganization of the CH domains is also confirmed by other crystal structures of utrophin, dystrophin, plectin and α-actinin[19–22].
The binding sites of plastin ABD1 on actin are located in two different subdomains (2 and 1) of the actin molecule[23]. The crystal structure also suggests that the two ABDs have non-identical interactions with F-actin because they each expose different surfaces to the solvent. This suggestion is consistent with the two different actin affinities found in L-plastin[24], in AtFim1[25] and in S pombe Fim1[26], and with the finding that the same mutations in both ABDs have different phenotypes in budding yeast[27]. Very recently, evidence has been put forward indicating that ABD1 of T-plastin is not only involved in actin-bundling, but may also control actin turnover, stabilization and assembly, independently of its bundling capacity[28].
Plastins in other organisms
Using an actin affinity matrix to identify actin-binding proteins in the budding yeast Saccharomyces cerevisiae, the Sac6 protein was identified, which localizes with cytoplasmic actin cables and cortical actin patches[29]. Its actin regulatory role was confirmed when the gene encoding this protein was found to suppress an actin mutation[30]. Sac6p shows 43% and 36% identity with chicken plastin and human plastin, respectively. Mutant budding yeast cells lacking this gene display temperature sensitivity defects in growth, morphology, endocytosis and sporulation[31,32]. Surprisingly, overexpression of Sac6p is lethal[33]. This could be explained by competition with an essential actin-binding protein, or by titrating out some other essential factor for growth. Next to stabilizing actin filaments, yeast plastin also has a role in the polymerization of G-actin[34,35]. Adams and colleagues demonstrated a high degree of functional conservation between evolutionarily divergent plastins[36]. They demonstrated that human T- and L-plastin could both substitute for yeast plastin in a Sac6 null mutant, and restore functional defects. However, the third isoform, I-plastin, could not complement this temperature sensitive growth defect, illustrating the functional differences between human isoforms. The fission yeast Schizosaccharomyces pombe also contains a plastin homologue, called Fim1. It is not essential for viability but has a role in cell morphogenesis. In mitotic cells, Fim1 plays a role in formation of the actin ring during cytokinesis[26]. Dictyostelium discoideum plastin shows 48%–50% identity with human plastins, and localizes to cortical structures associated with cell surface extensions[37].
In the ciliate Tetrahymena, plastin is localized in the cleavage furrow bundle during cytokinesis of dividing cells[38]. This protein cross-links actin filaments in a calcium-insensitive manner[39,40]. Tetrahymena plastin has a higher affinity for actin than the other plastin forms[39].
At least 3 plastin-like proteins may exist in the plant model system Arabidopsis thaliana[25,41]. The AtFim1 isoform contains an additional 65 amino acids at its carboxy-terminal end, and is Ca2+ insensitive because of less conserved Ca2+-binding domains. AtFim1 inhibits Zea mays profilin-induced actin depolymerization in vitro and in vivo[25].
L-plastin is often used as a myeloid lineage protein marker in zebrafish (Danio rerio)[42]. Spatio-temporal expression of the L-plastin zebrafish homologue reveals a high level of conservation between zebrafish and mammals. Thus zebrafish constitutes an informative model system for the study of normal and anomalous human myelopoiesis[43].
Expression and localization
The three plastin isoforms share approximately 70% homology in their amino acid sequences but are encoded by three distinct genes located on chromosomes 3 (I-plastin), 13 (L-plastin) and X (T-plastin). Analysis of the exon–intron junction sequences of all three human plastin genes indicates that they evolved from a common ancestor[12] (our unpublished observations). Their tissue-specific expression is strictly regulated: L-plastin expression is controlled by its strong promoter, regulated by its upstream repressor and by steroid hormone receptors[44,45]; the T-plastin promoter has a weak basal activity, and expression may be controlled by upstream enhancer elements and by methylation of a CpG island[46].
Generally, plastins are located in focal adhesions, ruffling membranes, lammellipodia, filopodia, or in specialized surface structures with highly ordered microfilament bundles such as microvilli and stereocilia. Sometimes they co-localize with stress fibers.
In adherent macrophages, L-plastin co-localizes with actin in podosomes and filopodia, and also exhibits a punctuate distribution in the cytoplasm that overlaps with actin. L-plastin is constitutively phosphorylated, and phosphorylated plastin is concentrated in the insoluble cytoskeletal fraction[47]. Overexpression of T- or L-plastin in a fibroblast-like cell line induces cell rounding and simultaneous actin stress fiber rearrangements. Both proteins promoted a reduction in the number and size of focal contacts in comparison to untransfected cells. In polarized epithelial cells, overexpression of T-plastin increases the length and density of microvilli. Because both isoforms can associate with different actin structures, they may play different roles in actin filament organization in a cell type-specific fashion[48].
Plastin is often found in stereocilia. Thus actin bundles formed by plastin may also have a mechano-sensory function by transforming mechanical alterations in signals during chemosensory signal transduction. T-plastin and I-plastin, but not L-plastin, are expressed in rat cochlea auditory hair cell stereocilia[49]. During postnatal development of the rat organ of Corti, T-plastin is detected in the core of stereocilia from the early stages of hair cell differentiation, and its expression gradually increases in stereocilia as hair cells mature. However, T-plastin is absent from mature hair cell stereocilia. In contrast, I-plastin is expressed in stereocilia and the cuticular plate from the early stages of hair cell differentiation[50] up to the adult stage[51–53]. The expression pattern of T-plastin in hair cell stereociliary bundles seems to be related to the location-specific length of hair cell stereocilia along the cochlear duct. Such temporally restricted expression strengthens the idea of functional differences between plastin isoforms, and suggests that T-plastin could have a specific role in sterocilia formation. Furthermore, plastin is also found in the microvilli of chicken photoreceptors[54], in microvillar projections in taste receptor cells of the mammalian taste bud[55], in microvilli from rat vomeronasal sensory epithelium[56], and in brush cells of the alimentary and respiratory system[57,58].
In general, it can be concluded that members of the plastin family have been identified in cellular regions containing polarized actin filaments, and in regions with a high actin filament turnover.
During differentiation of mouse intestine epithelium, three plastin isoforms are expressed in a cell-specific manner[59]. T- and L-plastin are present during the early stages of intestinal epithelial cell differentiation and localize to the apical and basal surface, respectively, until day 14.5, but disappear after day 16.5. I-plastin is first detected from day 14.5 at the apical surface. These findings suggest that plastin isoforms play different roles during epithelial cell differentiation. T- and I-plastin expression could be decisive for the formation and extension of the microvilli, whereas expression of L-plastin might play a role in controlling cell adhesion.
Because all plastin isoforms regulate the actin cytoskele-ton, they have always been considered as cytoplasmic proteins. Very recently, however, we reported that endo-genous as well as overexpressed T- and L-plastin were able to shuttle between nucleus and cytoplasm in HeLa and Jurkat cells. In most cells investigated, T-plastin is found exclusively in the cytoplasm, whereas L-plastin is located in the nucleus and cytoplasm[60]. We identified a strong leucine-rich nuclear export signal (NES) in T-plastin that was less conserved in L-plastin. This is due, in part, to lack of a phenylalanine residue in the weak NES of L-plastin. This particular residue forms part of the core amino acids that constitute the strong NES of T-plastin. When phenylalanine is inserted into the NES of L-plastin, we observed enhanced export activity of L-plastin from the nucleus. It is likely that shuttling occurs in other cell types as well, but this has not yet been demonstrated. The functional relevance of this nucleo-cytoplasmic shuttling of T- and L-plastin remains unclear at present. L-plastin could be implicated in the regulation of nuclear actin. Indeed, actin is an essential component of the pre-initiation complex and cooperates with polymerase I, II, and III in gene expression[61–63].
Regulation of plastin function
Calcium The two calmodulin-like calcium-binding domains in plastins, the so-called EF-hands, suggest that calcium could regulate actin-binding or other functions of plastins. The rather weak homology in the calcium binding domains of plastin isoforms may suggest that their actin-binding activities are differentially regulated by calcium. Human L-plastin is the only isoform that possesses all the conserved amino acids essential for calcium binding and, indeed, human L-plastin bundles actin filaments in a strictly calcium-regulated manner. Bundles are formed at pCa 7, but not at pCa 6 (free calcium concentration)[64]. I-plastin bundling activity is also inhibited by calcium[13]. The calcium sensitivity of T-plastin has not been studied thoroughly in the past, although some reports mention this[65,23,66]. Recently, this aspect was investigated by Giganti and colleagues, who showed that co-sedimentation of actin with T-plastin was not affected by free calcium concentrations of up to 2.2 µmol/L, in contrast to L-plastin activity, which was inhibited by calcium[28]. Similarly, in an F-actin depolymerization assay, no effect of T-plastin was observed in a free calcium concentration range of 4.6 nmol/L–1.6 µmol/L. Strangely, yeast plastin, Sac6p, is regulated by Ca2+, although it does not contain the conserved residues required for calcium binding[35]. The Tetrahymena plastin, Arabidopsis Atfim1 and fission yeast Fim1 bundle actin filaments in a calcium-insensitive manner[65,26,39]. In contrast, Dictyostelium discoideum plastin bundles actin in a calcium-sensitive manner[37].
Phospholipids Using solid-phase immunoassays it was found that increasing amounts of phospholipids affected the binding between monomeric actin and L-plastin. In the presence of PIP2, and to a smaller extent phosphatidylinositol, the level of actin-L-plastin interaction decreases steadily. The observed inhibitory effect reaches 50% at 50 µg/mL PIP2. However, diacylglycerol did not produce such an effect[24]. It remains to be demonstrated if this also applies to other plastin isoforms, and if the plastin-F-actin interaction would also be affected. Nevertheless, these findings may suggest that plastin activity is also susceptible to regulation by phospholipids, and this would tie in with many reports detailing modulation of the cytoskeleton through phospholipid interaction with actin-binding proteins.
Phosphorylation Only L-plastin has been reported to be phosphorylated in vitro and in vivo. Phosphorylation occurs particularly in hematopoietic cells, although this modification may also take place in malignant cells that express L-plastin. Two amino-terminally located serine residues, Ser5 and Ser7, are involved[67]. Protein kinase A (PKA) can phosphorylate plastin in vitro[68,69]. In T-plastin, the Ser-7 residue is conserved, but has never been observed to be phosphorylated.
Ser5 is phosphorylated in HeLa cells. It is possible that Ser 7 is also phosphorylated but only after phosphorylation of Ser 5[70]. Another intensive study on L-plastin phosphorylation was done by Lin and colleagues[71]. They used fibroblasts stably transfected with wild type plastin and mutant plastins, in which each of the serines were mutated. Only the wild type was phosphorylated, but not the mutants, pointing to a possible phosphorylation zipper mechanism. All the data available so far point out that phosphorylation of L-plastin involves a complex mechanism that is not yet completely understood. L-plastin phosphorylation is also dependent on cell type and stimulus. Phosphorylation may regulate actin-binding/bundling, or interactions between plastin and other proteins.
Binding partners
Apart from actin, there have not been many plastin-binding partners characterized yet. However, L-plastin has been reported to be in complex with several proteins in hematopoietic cells. Grancalcin is a Ca2+-binding protein, which is abundant in human neutrophils. Both proteins interact in hematopoietic cells and their interaction is negatively regulated by Ca2+ [72]. In adherent macrophages, L-plastin binds vimentin and Hsp70[73]. Only the L-plastin-vimentin interaction was further characterized. Plastin forms an adhesion-dependent complex with a vimentin subunit tetramer, but not with vimentin intermediate filaments. Both proteins interact with an affinity of 0.25 µmol/L (Kd) and co-localize in podosomes, filopodia and retraction fibers. Co-localization in or around the nucleus was also occasionally observed. The amino-terminal domain of vimentin was identified as the plastin-binding site, and the vimentin-binding site localizes to the first CH domain of plastin. Phosphorylation of plastin is probably not involved in complex formation. The interaction may play a role in directing the assembly of the vimentin cytoskeleton at cell adhesion sites.
L-plastin also interacts with Iba1, a microglia/macrophage-specific calcium-binding and actin-bundling protein[74]. In response to stimuli, L-plastin co-localizes with Iba1 and F-actin in membrane ruffles and phagocytic cups. The interaction is direct and independent of Ca2+. Furthermore, Iba1 increases the actin-bundling activity of L-plastin.
Cellular functions of plastin isoforms
Phosphorylation and leukocyte function Leukocyte activation is an important aspect of inflammation and immunity. Quite a number of studies have addressed the phosphorylation of L-plastin during the activation of hematopoietic cells in response to a variety of signals.
In polymorphonuclear (PMN) leukocytes, L-plastin phosphorylation is induced by IL-1 after glucocorticoid treatment and this is blocked by inhibitors of adenylyl cyclase and protein kinase A, but not by a PKC inhibitor[75]. In stimulated cells, phosphorylation of L-plastin increases from 5% to 30%[68]. TNF treatment of peripheral blood mononuclear cells also induces the phosphorylation of L-plastin, but activators of protein kinase A fail to increase phosphorylation, suggesting that other kinases could be involved[76]. IL-8 and the chemotactic peptide fMLP (both chemoattractants for PMN) also promote phosphorylation of L-plastin. In this study, phosphorylation was inhibited by PKA inhibitors, whereas phorbol 12-myristate 13-acetate (PMA, a direct activator of PKC) stimulated phosphorylation significantly[77]. However, there is little evidence to support L-plastin phosphorylation by PKC in vivo. Nevertheless, these few examples illustrate that several stimulatory signals induce phosphorylation of L-plastin through distinct pathways in which PKA can sometimes act as a direct kinase, with PKC acting as an indirect effector. Bacterial lipopolysaccharide (LPS) also induces phosphorylation of L-plastin in murine peritoneal macrophages[78]. Phosphorylation closely correlated with cellular responses such as the production of inflammato-genic substances such as TNF, IL-1 and arachidonic acid, a precursor for prostaglandin synthesis in T-lymphocytes. Phosphorylation of L-plastin was discovered as an accessory receptor-mediated co-stimulatory event[79].
Apart from the phosphorylation of L-plastin induced by chemoattractants, interleukins, PMA or fMLP in various hematopoietic cells, L-plastin function and phosphorylation in leukocyte activation was also studied by ligation of IgGFc receptors. Ligation of FcγR in PMN by immune complexes leads to several effector events, including the secretion of inflammatory cytokines and vasoactive lipids, phagocytosis, antibody-dependent cell cytotoxicity and a respiratory burst[80]. Early events following ligation also include the polymerization of actin. More specifically, FcγRII adhesion and phagocytosis of IgG-opsonized particles induces L-plastin phosphorylation and L-plastin localization to the podosomes. L-plastin phosphorylation is not blocked by cytochalasin D[81], suggesting that phosphorylation does not require an intact actin cytoskeleton.
Integrin aMb2 (CD18) activation in PMN is necessary for sustained adhesion and involves L-plastin phosphoryl-ation. To find out if the N-terminal region of L-plastin is sufficient for regulating adhesion, L-plastin-derived (mutant) peptides (amino acids 2–19) were used in PMN following fusion with the Tat peptide[70]. These studies showed that wild type and the Ser5-phosphorylated peptide induced adhesion to serum-coated surfaces, but not activation of the respiratory burst or an increase in [Ca2+]ic. A corresponding T-plastin peptide or the L-plastin Ser5/Ala mutant peptide were inactive. Strangely, the activating peptides also induce phosphorylation of endogenous L-plastin. Phosphorylation could be blocked by PKC and PI3K inhibitors, but again L-plastin could not be phosphorylated in vitro by PKC.
Interestingly, PMN leukocytes lacking L-plastin are defective for killing pathogens in vivo and in vitro, even though they show normal migration to the site of infection, adhesion and spreading, and normal phagocytosis. Although L-plastin phosphorylation is known to be implicated in integrin activation[70,82], it seems not to be required here. L-plastin is involved in activation of the adhesion-dependent respiratory burst by signaling to NADPH oxidase[83]. L-plastin-deficient PMN cells had no altered morphology, which could be due to redundancy in actin-binding proteins.
Anti-leukoproteinase is a physiological inhibitor of granulocytic serine proteases. Anti-protease treatment reduces the incidence and severity of arthritis, and has a protective effect against cartilage and bone erosion. Anti-leukoproteinase binds to L-plastin and downregulates filamentous actin assembly in response to stimulation with IgG-coated latex beads in granulocytes. Anti-leukoproteinase also exerts additional inhibitory effects on neutrophil functions, such as phagocytosis and oxidative bursts[84]. Thus anti-leukoproteinase has anti-arthritis potential because of the modulation of L-plastin in neutrophils.
A role for T-plastin during invasion of bacteria T-plastin is involved in invasion by at least two enteropathic bacteria: Shigella flexneri and Salmonella typhimurium. These two bacteria have different mechanisms for entering non-phagocytic cells by using specific effector proteins. In both cases, T-plastin is involved in cytoskeletal rearrangements during bacterial invasion. These rearrangements consist of distinct nucleation zones involving strong actin polymerization in close proximity to the contact site between bacterium and cell. These structures then push cellular protrusions outward that engulf the entering bacterium. T-plastin is concentrated in the protrusions during invasion of Shigella flexneri[85,86]. Through transfection experiments it can be shown that T- and L-plastin are differentially recruited into Shigella entry zones, reflecting their distinct binding specificities. Transient expression of a dominant negative truncated T-plastin mutant decreased Shigella entry by 64%, indicating that T-plastin has a functional role in Shigella entry into HeLa cells[85].
During Salmonella typhimurium invasion, T-plastin is first recruited to membrane ruffles induced by the bacterium via a Cdc42-dependent signaling process that is activated by the bacterial secreted protein SopE. Then another secreted bacterial effector protein, SipA, forms a complex with T-plastin and F-actin, which results in a marked increase in the actin-bundling activity of T-plastin. SipA also inhibits actin depolymerization. This leads to stabilization of actin filaments at the point of bacterium-host cell contact, which leads to more efficient Salmonella internalization[66].
Plastins and DNA repair Several independent reports indicate that plastin is involved in the cellular response to DNA-damaging agents and toxins. First of all, T-plastin expression is enhanced in cisplatin-resistant human bladder, prostatic, head and neck cancer cell lines, in comparison to their cisplatin-sensitive counterparts. Cisplatin is an anticancer agent that acts by binding to DNA and interfering with DNA repair. T-plastin mRNA is 12-fold more abundant in cisplatin-resistant cells in comparison to parental cells[87]. Furthermore, T-plastin is also upregulated in UV radiation-resistant cells[88]. In addition, downregulation of T-plastin expression is associated with increased sensitivity to cispla-tin[87]. Increased expression of T-plastin has been observed in Chinese hamster ovary (CHO) cells in which G2 arrest has been induced by X-radiation and by a topoisomerase II inhibitor, etoposide[89]. In contrast, when T-plastin expression was downregulated, radiation-induced G2 arrest decreased in CHO cells, indicating a correlation between T-plastin expression and G2/M cell-cycle control.
Vinca alkaloids such as vincristin and vinblastin are chemotherapeutic agents used in the treatment of both childhood and adult cancers. Their main cellular target is the β-tubulin subunit of α/β-tubulin heterodimers, and they inhibit cell division by disrupting microtubule dynamics. Interestingly, L-plastin is downregulated in response to the vincristin treatment of drug-sensitive human leukemia cells and in vinblastin-resistant cells[90]. These alterations in expression could be involved in several protector functions specific for each drug. For example, T-plastin could play a role in alteration of the intracellular distribution of the drug[87]. Alternatively, the drug might be trapped in the cytoplasm by plastin-associated actin. T-plastin could also have a direct or indirect role in DNA repair. In UV radiation-resistant cells, this last possibility is a good candidate because these cells have a greater capacity to repair DNA[91]. Furthermore, T-plastin expression is suppressed in a human colorectal cancer cell line, SW948, because of promoter-specific DNA methylation[89], which might be explained by T-plastin’s possible role in checkpoint function. Taking everything into consideration, it seems likely that the protecting role of plastin is due to its involvement in DNA repair. Our finding that plastins can shuttle between nucleus and cytoplasm may strengthen this hypothesis[60].
Cancer A very interesting and uncommon finding is that L-plastin is specifically expressed in many transformed cells but absent in their normal cell counterparts[6,7]. Indeed, L-plastin has been described as a marker for many human cancer cells of non-hematopoietic origin[92]. Lin et al found that 68% of epithelial carcinomas investigated and 53% of non-epithelial mesenchymal tumors examined expressed L-plastin (remember that L-plastin is normally expressed only in cells of the hematopoietic lineage)[12]. In addition, examination of human neoplastic cell lines revealed that more than 90% of the cell lines surveyed exhibited widely varying degrees of L-plastin expression[93]. In particular, cells derived from the reproductive tract expressed L-plastin. In normal cells of reproductive tissues (which are responsive to ovarian steroids), L-plastin synthesis is induced by female hormones. During malignancy, expression becomes hormone-independent[94]. The L-plastin promoter harbors several hormone receptor-responsive elements; that is, one estrogen-responsive element and three imperfect androgen-responsive elements[44,45]. Estrogen receptor binding[95] and cooperative androgen receptor binding have been demonstrated[45].
In 1997, Zheng and colleagues[96] found that expression of L-plastin in prostatic epithelial cells was linked to the malignant state. Using antisense L-plastin constructs in prostate carcinoma cell lines (PC-3 and PC-3M cells, the metastatic variant), it was demonstrated that cell proliferation and invasion were drastically reduced[97].
Because L-plastin expression is generally considered to be a marker for many cancers[92], new therapeutic tools have been developed aimed at slowing down cancer progression in vivo based on the L-plastin gene. Gene therapy experiments in an animal model of colon cancer[98,99] involving a pro-drug approach have shown promising results: the cytosine deaminase gene, driven by an L-plastin tumor-specific promoter, modifies 5-fluorocytosine into a toxic derivative, fluorouracil. These vectors induced significant toxicity in carcinomas of the breast, ovary and colon in vitro and in vivo: tumor size as well as tumor cell growth decreased significantly. Mice injected with the expression vector and 5-fluorocytosine lived much longer than their untreated litter mates[98,99].
Although these approaches are promising, we still lack fundamental molecular data showing how increased (or ectopic) plastin expression contributes to tumor formation. In the same line, a clear causal relationship between plastin expression and invasion/metastasis, the hallmarks of malignant tumors, needs to be established and worked out at the molecular level. This will allow models to be put forward and thoroughly tested in vitro and in animal models. These approaches could help resolve the question of whether plastins could be valuable targets for drug development in the treatment of cancer.
Other diseases In an animal model of the auto-immune disease systemic lupus erythematosus (SLE, a chronic rheumatic disease) and in human patients, antibodies against T- and L-plastin were found in serum[100,101,102]. The presence of T- and L-plastin antibodies is correlated with the presence of the anti-Sm antibody, a typical SLE auto-antibody that recognizes a nuclear antigen. The stimulus for production of L- and T-plastin auto-antibodies may derive from the destruction of plastin-containing cells, such as replicating white blood cells (L-plastin), or epithelial and mesenchymal surfaces (T-plastin). It remains to be determined if L- and T-plastin antibodies contribute to the loss of cell function or other aspects of lupus. L-plastin has also been identified as a self-antigen associated with Vogt-Koyanagi’s syndrome, an autoimmune disease[103].
Expression of a chimeric mRNA transcript between the LAZ3 gene and the L-plastin gene, resulting from chromosomal translocation, was observed in two B-cell non-Hodgkin-lymphomas. The 13q14 chromosome region, where the L-plastin gene is located, is frequently disrupted in various proliferative disorders, and defines a breakpoint site[104].
Finally, minimal change nephrotic syndrome (MCNS) is the most frequent glomerular disease in children, and is characterized by heavy proteinuria[105]. MCNS results from a systemic disorder of T cell function[106]. Expression levels of L-plastin and grancalcin are increased during the period of disease relapse[107]. A truncated protein, Tc-mip, was detected in peripheral blood mononuclear cells from MCNS patients. Overexpression of this truncated protein in T cell Jurkat cells induced redistribution of L-plastin, in addition to Src phosphorylation and T cell clustering[108]. Increased expression and redistribution of L-plastin may result in T-cell dysfunction, causing MCNS. These fragmentary data suggest that plastins contribute to the onset or progression of certain diseases, but it is clear that much more work needs to be done to ascertain their true role in these syndromes.
Concluding remarks and future directions
Although plastins have a clear role in actin-binding, there is sometimes apparently incongruent information as to their exact actin-binding properties. Actin-bundling activity by mammalian plastins has been clearly established, but there is additional evidence for actin filament stabilization[26] and for filament anti-depolymerization activities[109,24,28,27]. In some organisms (yeast and plants), there is even indication that plastin has actin polymerization ability[35,25].
The depletion of plastin does not promote severe deficiencies at first glance, because the knockout of L-plastin has no influence on embryonic and neonatal development[83]. However, a lack of L-plastin could lead to immunological problems in a broad sense or complications involving inflam-mation.
Some culture cells overexpressing T- or L-plastin loose their adhesion properties and round up[48,110]. It is hard to generate stable cell lines overexpressing plastin[71,93], and this could be related to the observed invasive behavior of tumor cells (over)expressing plastin.
Future studies are expected to provide more clarity with respect to the functional significance of cell-type-specific expression of the three human plastin isoforms, each with its individual properties and functional regulation. In addition, nucleocytoplasmic shuttling of plastin isoforms elicits the question of what their true function in the nucleus is.
Acknowledgements
This work was supported by the Belgian Federation Against Cancer, the Fund for Scientific Research-Flanders (FWO-Vlaanderen), the IUAP and Fortis Bank Verzekeringen. VD is supported by a PhD grant from the Institute for the Promotion of Innovation through Science and Technology in Flanders (IWT-Vlaanderen).
We apologize to those colleagues whose work has not been mentioned in the text.
References
- Dos Remedios CG, Chhabra D, Kekic M, Dedova IV, Tsubakihara M, Berry DA, et al. Actin binding proteins: Regulation of cytoskeletal microfilaments. Physiol Rev 2003;83:433-73.
- Matsudaira PT, Burgess DR. Identification and organization of the components in the isolated microvillus cytoskeleton. J Cell Biol 1979;83:667-73.
- Bretscher A, Weber K. Fimbrin, a new microfilament-associated protein present in microvilli and other cell-surface structures. J Cell Biol 1980;86:335-40.
- Bretscher A. Fimbrin is a cytoskeletal protein that crosslinks F-actin in vitro. Proc Natl Acad Sci USA 1981;78:6849-53.
- Glenney JR, Kaulfus P, Matsudaira P, Weber K. F-Actin binding and bundling properties of fimbrin, a major cytoskeletal protein of microvillus core filaments. J Biol Chem 1981;256:9283-8.
- Leavitt J, Kakunaga T. Expression of a variant form of actin and additional polypeptide changes following chemical induced in vitro neoplastic transformation of human fibroblasts. J Biol Chem 1980;255:1650-61.
- Leavitt J, Goldman D, Merril C, Kakunaga T. Changes in gene expression accompanying chemically induced malignant transformation of human fibroblasts. Carcinogenesis 1982;3:61-70.
- Goldstein D, Djeu J, Latter G, Burbeck S, Leavitt J. Abundant synthesis of the transformation induced protein of neoplastic human fibroblasts, plastin, in normal lymphocytes. Cancer Res 1985;45:5643-7.
- Lin CS, Aebersold RH, Kent SB, Varma M, Leavitt J. Molecular cloning and characterization of plastin, a human leukocyte protein expressed in transformed human fibroblasts. Mol Cell Biol 1988;8:4659-68.
- Lin CS, Aebersold RH, Leavitt J. Correction of the N-terminal sequences of the human plastin isoforms by using anchored polymerase chain-reaction: identification of a potential calcium-binding domain. Mol Cell Biol 1990;10:1818-21.
- Zu YL, Shigesada K, Nishida E, Kubota I, Kohno M, Hanaoka M, et al. 65-Kilodalton protein phosphorylated by interleukin-2 stimulation bears 2 putative actin-binding sites and 2 calcium-binding sites. Biochemistry 1990;29:8319-24.
- Lin CS, Park T, Chen ZP, Leavitt J. Human plastin genes. Comparative gene structure, chromosome location, and differential expression in normal and neoplastic cells. J Biol Chem 1993;268:2781-92.
- Lin CS, Shen WY, Chen ZP, Tu YH, Matsudaira P. Identification of I-plastin, a human fimbrin isoform expressed in intestine and kidney. Mol Cell Biol 1994;14:2457-67.
- De Arruda MV, Watson S, Lin CS, Leavitt J, Matsudaira P. Fimbrin is a homologue of the cytoplasmic phosphoprotein plastin and has domains homologous with calmodulin and actin gelation proteins. J Cell Biol 1990;111:1069-79.
- Gimona M, Djinovic-Carugo K, Kranewitter WJ, Winder SJ. Functional plasticity of CH domains. FEBS Lett 2002;513:98-106.
- Korenbaum E, Rivero F. Calponin homology domains at a glance. J Cell Sci 2002;115:3543-5.
- Klein MG, Shi WX, Ramagopal U, Tseng Y, Wirtz D, Kovar DR, et al. Structure of the actin crosslinking core of fimbrin. Structure 2004;12:999-1013.
- Goldsmith SC, Pokala M, Shen WY, Fedorov AA, Matsudaira P, Almo SC. The structure of an actin-crosslinking domain from human fimbrin. Nat Struct Biol 1997;4:708-12.
- Garcia-Alvarez B, Bobkov A, Sonnenberg A, de Pereda JM. Structural and functional analysis of the actin binding domain of plectin suggests alternative mechanisms for binding to F-actin and integrin beta 4. Structure 2003;11:615-25.
- Keep NH, Winder SJ, Moores CA, Walke S, Norwood FLM, Kendrick-Jones J. Crystal structure of the actin-binding region of utrophin reveals a head-to-tail dimer. Structure Fold Des 1999;7:1539-46.
- Liu J, Taylor DW, Taylor KA. A. 3-D reconstruction of smooth muscle alpha-actinin by CryoEm reveals two different conformations at the actin-binding region. J Mol Biol 2004;338:115-25.
- Norwood FLM, Sutherland-Smith AJ, Keep NH, Kendrick-Jones J. The structure of the N-terminal actin-binding domain of human dystrophin and how mutations in this domain may cause Duchenne or Becker muscular dystrophy. Structure Fold Des 2000;8:481-91.
- Hanein D, Volkmann N, Goldsmith S, Michon AM, Lehman W, Craig R, et al. An atomic model of fimbrin binding to F-actin and its implications for filament crosslinking and regulation. Nat Struct Biol 1998;5:924.
- Lebart MC, Hubert F, Boiteau C, Venteo S, Roustan C, Benyamin Y. Biochemical characterization of the L-plastin-actin interaction shows a resemblance with that of alpha-actinin and allows a distinction to be made between the two actin-binding domains of the molecule. Biochemistry 2004;43:2428-37.
- Kovar DR, Staiger CJ, Weaver EA, McCurdy DW. AtFim1 is an actin filament crosslinking protein from Arabidopsis thaliana. Plant J 2000;24:625-36.
- Nakano K, Satoh K, Morimatsu A, Ohnuma M, Mabuchi I. Interactions among a fimbrin, a capping protein, and an actin-depolymerizing factor in organization of the fission yeast actin cytoskeleton. Mol Biol Cell 2001;12:3515-26.
- Brower SM, Honts JE, Adams AEM. Genetic-analysis of the fimbrin-actin binding interaction in Saccharomyces cerevisiae. Genetics 1995;140:91-101.
- Giganti A, Plastino J, Janji B, Van Troys M, Lentz D, Ampe C, et al. Actin-filament cross-linking protein T-plastin increases Arp2/3-mediated actin-based movement. J Cell Sci 2005;118:1255-65.
- Drubin DG, Miller KG, Botstein D. Yeast actin-binding proteins: Evidence for a role in morphogenesis. J Cell Biol 1988;107:2551-61.
- Adams AEM, Botstein D, Drubin DG. A yeast actin-binding protein is encoded by sac6, a gene found by suppression of an actin mutation. Science 1989;243:231-3.
- Kubler E, Riezman H. Actin and fimbrin are required for the internalization step of endocytosis in yeast. Embo J 1993;12:2855-62.
- Adams AEM, Botstein D, Drubin DG. Requirement of yeast fimbrin for actin organization and morphogenesis in vivo. Nature 1991;354:404-8.
- Sandrock TM, Brower SM, Toenjes KA, Adams AEM. Suppressor analysis of fimbrin (Sac6p) overexpression in yeast. Genetics 1999;151:1287-97.
- Cheng DM, Rubenstein PA. Interaction of yeast fimbrin (Sac6p) with a polymerization-defective (GG) actin. Mol Biol Cell 1998;9:269A.
- Cheng DM, Marner J, Rubenstein PA. Interaction in vivo and in vitro between the yeast fimbrin, SAC6P, and a polymerization defective yeast actin (V266G and L267G). J Biol Chem 1999;274:873-80.
- Adams AEM, Shen WY, Lin CS, Leavitt J, Matsudaira P. Isoform specific complementation of the yeast Sac6 null mutation by human fimbrin. Mol Cell Biol 1995;15:69-75.
- Prassler J, Stocker S, Marriott G, Heidecker M, Kellermann J, Gerisch G. Interaction of a Dictyostelium member of the plastin/fimbrin family with actin filaments and actin-myosin complexes. Mol Biol Cell 1997;8:83-95.
- Watanabe A, Kurasawa Y, Watanabe Y, Numata O. A new Tetrahymena actin-binding protein is localized in the division furrow. J Biochem 1998;123:607-13.
- Shirayama S, Numata O. Tetrahymena fimbrin localized in the division furrow bundles actin filaments in a calcium-independent manner. J Biochem 2003;134:591-8.
- Watanabe A, Yonemura I, Gonda K, Numata O. Cloning and sequencing of the gene for a Tetrahymena fimbrin-like protein. J Biochem 2000;127:85-94.
- McCurdy DW, Kim M. Molecular cloning of a novel fimbrin-like cDNA from Arabidopsis thaliana. Plant Mol Biol 1998;36:23-31.
- Herbomel P, Thisse B, Thisse C. Ontogeny and behaviour of early macrophages in the zebrafish embryo. Development 1999;126:3735-45.
- Bennett CM, Kanki JP, Rhodes J, Liu TX, Paw BH, Kieran MW, et al. Myelopoiesis in the zebrafish, Danio rerio. Blood 2001;98:643-51.
- Lin CS, Chen ZP, Park T, Ghosh K, Leavitt J. Characterization of the human L-plastin gene promoter in normal and neoplastic cells. J Biol Chem 1993;268:2793-801.
- Lin CS, Lau A, Yeh CC, Chang CH, Lue TF. Upregulation of L-plastin gene by testosterone in breast and prostate cancer cells: identification of three cooperative androgen receptor-binding sequences. DNA Cell Biol 2000;19:1-7.
- Lin CS, Lau A, Huynh T, Lue TF. Differential regulation of human T-plastin gene in leukocytes and non-leukocytes: identification of the promoter, enhancer, and CpG island. DNA Cell Biol 1999;18:27-37.
- Messier JM, Shaw LM, Chafel M, Matsudaira P, Mercurio AM. Fimbrin localized to an insoluble cytoskeletal fraction is constitutively phosphorylated on its headpiece domain in adherent macrophages. Cell Motil Cytoskeleton 1993;25:223-33.
- Arpin M, Friederich E, Algrain M, Vernel F, Louvard D. Functional differences between L-plastin and T-plastin isoforms. J Cell Biol 1994;127:1995-2008.
- Daudet N, Lebart MC. Transient expression of the T-isoform of plastin/fimbrin in the stereocilia of developing auditory hair cells. Cell Motil Cytoskeleton 2002;53:326-36.
- Zine A, Hafidi A, Romand R. Fimbrin expression in the developing rat cochlea. Hearing Res 1995;87:165-9.
- Flock A, Bretscher A, Weber K. Immunohistochemical localization of several cytoskeletal proteins in inner-ear sensory and supporting cells. Hearing Res 1982;7:75-89.
- Sobin A, Flock A. Immunohistochemical identification and localization of actin and fimbrin in vestibular hair cells in the normal guinea-pig and in a strain of the Waltzing guinea-pig. Acta Otolaryngol 1983;96:407-12.
- Slepecky N, Chamberlain SC. Immunoelectron microscopic and immunofluorescent localization of cytoskeletal and muscle like contractile proteins in inner-ear sensory hair cells. Hearing Res 1985;20:245-60.
- Hofer D, Drenckhahn D. Molecular heterogeneity of the actin filament cytoskeleton associated with microvilli of photo-receptors, Muller glial-cells and pigment epithelial-cells of the retina. Histochemistry 1993;99:29-35.
- Hofer D, Drenckhahn D. Localisation of actin, villin, fimbrin, ezrin and ankyrin in rat taste receptor cells. Histochem Cell Biol 1999;112:79-86.
- Hofer D, Shin DW, Drenckhahn D. Identification of cytoskeletal markers for the different microvilli and cell types of the rat vomeronasal sensory epithelium. J Neurocytol 2000;29:147-56.
- Hofer D, Drenckhahn D. Identification of brush cells in the alimentary and respiratory system by antibodies to villin and fimbrin. Histochem 1992;98:237-42.
- Hofer D, Drenckhahn D. Cytoskeletal markers allowing discrimination between brush cells and other epithelial cells of the gut including enteroendocrine cells. Histochem Cell Biol 1996;105:405-12.
- Chafel MM, Shen WY, Matsudaira P. Sequential expression and differential localization of I-fimbrin, L-fimbrin, and T-fimbrin during differentiation of the mouse intestine and yolk-sac. Dev Dyn 1995;203:141-51.
- Delanote V, Van Impe K, De Corte V, Bruyneel E, Vetter G, Boucherie C, et al. Molecular basis for dissimilar nuclear trafficking of the actin-bundling protein isoforms T- and L-plastin. Traffic 2005;6:335-45.
- Hofmann WA, Stojiljkovic L, Fuchsova B, Vargas GM, Mavrommatis E, Philimonenko V, et al. Actin is part of pre-initiation complexes and is necessary for transcription by RNA polymerase II. Nat Cell Biol 2004;6:1094-101.
- Hu P, Wu S, Hernandez N. A role for beta-actin in RNA polymerase III transcription. Genes Dev 2004;18:3010-5.
- Philimonenko V, Zhao J, Iben S, Dingova H, Kysela K, Kahle M, et al. Nuclear actin and myosin I are required for RNA polymerase I transcription. Nat Cell Biol 2004;6:1165-72.
- Namba Y, Ito M, Zu YL, Shigesada K, Maruyama K. Human T-cell L-plastin bundles actin-filaments in a calcium-dependent manner. J Biochem 1992;112:503-7.
- Kovar DR, Gibbon BC, McCurdy DW, Staiger CJ. Fluorescently-labeled fimbrin decorates a dynamic actin filament network in live plant cells. Planta 2001;213:390-5.
- Zhou DG, Mooseker MS, Galan JE. An invasion-associated Salmonella protein modulates the actin-bundling activity of plastin. Proc Natl Acad Sci USA 1999; 96: 10 176–81.
- Shinomiya H, Hagi A, Fukuzumi M, Mizobuchi M, Hirata H, Utsumi S. Complete primary structure and phosphorylation site of the 65-kDa macrophage protein phosphorylated by stimulation with bacterial lipopolysaccharide. J Immunol 1995;154:3471-8.
- Matsushima K, Shiroo M, Kung HF, Copeland TD. Purification and characterization of a cytosolic 65-kilodalton phosphoprotein in human-leukocytes whose phosphorylation is augmented by stimulation with interleukin-1. Biochemistry 1988;27:3765-70.
- Wang J, Brown EJ. Immune complex-induced integrin activation and L-plastin phosphorylation require protein kinase A. J Biol Chem 1999; 274: 24 349–56.
- Jones SL, Wang J, Turck CW, Brown EJ. A role for the actin-bundling protein L-plastin in the regulation of leukocyte integrin function. Proc Natl Acad Sci USA 1998;95:9331-6.
- Lin CS, Lau A, Lue TF. Analysis and mapping of plastin phosphorylation. DNA Cell Biol 1998;17:1041-6.
- Lollike K, Johnsen AH, Durussel I, Borregaard N, Cox JA. Biochemical characterization of the penta-EF-hand protein grancalcin and identification of L-plastin as a binding partner. J Biol Chem 2001;276:17762-9.
- Correia I, Chu D, Chou YH, Goldman RD, Matsudaira P. Integrating the actin and vimentin cytoskeletons: adhesion-dependent formation of fimbrin-vimentin complexes in macrophages. J Cell Biol 1999;146:831-42.
- Ohsawa K, Imai Y, Sasaki Y, Kohsaka S. Microglia/macrophage-specific protein Iba1 binds to fimbrin and enhances its actin-bundling activity. J Neurochem 2004;88:844-56.
- Matsushima K, Kobayashi Y, Copeland TD, Akahoshi T, Oppenheim JJ. Phosphorylation of a cytosolic 65-kDa protein, induced by interleukin-1 in glucocorticoid pretreated normal human peripheral-blood mononuclear leukocytes. J Immunol 1987;139:3367-74.
- Shiroo M, Matsushima K. Enhanced phosphorylation of 65 and 74 kDa proteins by tumor necrosis factor and interleukin-1 in human peripheral blood mononuclear cells. Cytokine 1990;2:13-20.
- Shibata M, Yamakawa Y, Ohoka T, Mizuno S, Suzuki K. Characterization of a 64-kD protein phosphorylated during chemotactic activation with IL-8 and fMLP of human polymorphonuclear leukocytes . Purification and aminoacid analysis of phosphorylated 64-kD protein. J Leukoc Biol 1993;54:10-6.
- Shinomiya H, Hirata H, Nakano M. Purification and characterization of the 65-kD protein phosphorylated in murine macrophages by stimulation with bacterial lipopolysaccharide. J Immunol 1991;146:3617-25.
- Henning SW, Meuer SC, Samstag Y. Serine phosphorylation of a 67-kD protein in human T-lymphocytes represents an accessory receptor-mediated signaling event. J Immunol 1994;152:4808-15.
- Schubert J, Hundt M, Schmidt R. Fc gamma receptor mediated activation of human polymorphonuclear neutrophils (PMN). Arch Immunol Ther Exp 1992;40:65-6.
- Jones SL, Brown EJ. Fc gamma RII-mediated adhesion and phagocytosis induce L-plastin phosphorylation in human neutrophils. J Biol Chem 1996;271:14623-30.
- Wang J, Chen H, Brown EJ. L-plastin peptide activation of alpha(v)beta(3)-mediated adhesion requires integrin conformational change and actin filament disassembly. J Biol Chem 2001;276:14474-81.
- Chen H, Mocsai A, Zhang H, Ding RX, Morisaki JH, White M, et al. Role for plastin in host defense distinguishes integrin signaling from cell adhesion and spreading. Immunity 2003;19:95-104.
- Sehnert B, Cavcic A, Bohm B, Kalden JR, Nandakumar KS, Holmdahl R, et al. Antileukoproteinase: modulation of neutrophil function and therapeutic effects on anti-type II collagen antibody-induced arthritis. Arthritis Rheum 2004;50:2347-59.
- Adam T, Arpin M, Prevost MC, Gounon P, Sansonetti PJ. Cytoskeletal rearrangements and the functional role of T-plastin during entry of Shigella flexneri into Hela-cells. J Cell Biol 1995;129:367-81.
- Prevost MC, Lesourd M, Arpin M, Vernel F, Mounier J, Hellio R, et al. Unipolar reorganization of F-actin layer at bacterial division and bundling of actin-filaments by plastin correlate with movement of Shigella flexneri within Hela cells. Infect Immun 1992;60:4088-99.
- Hisano T, Ono M, Nakayama M, Naito S, Kuwano M, Wada M. Increased expression of T-plastin gene in cisplatin-resistant human cancer cells: Identification by mRNA differential display. FEBS Lett 1996;397:101-7.
- Higuchi Y, Kita K, Nakanishi H, Wang XL, Sugaya S, Tanzawa H, et al. Search for genes involved in UV-resistance in human cells by mRNA differential display: Increased transcriptional expression of nucleophosmin and T-plastin genes in association with the resistance. Biochem Biophys Res Commun 1998;248:597-602.
- Sasaki Y, Itoh F, Kobayashi T, Kikuchi T, Suzuki H, Toyota M, et al. Increased expression of T-fimbrin gene after DNA damage in CHO cells and inactivation of T-fimbrin by CpG methylation in human colorectal cancer cells. Int J Cancer 2002;97:211-6.
- Verrills NM, Walsh BJ, Cobon GS, Hains PG, Kavallaris M. Proteome analysis of Vinca alkaloid response and resistance in acute lymphoblastic leukemia reveals novel cytoskeletal alterations. J Biol Chem 2003; 278: 45 082–93.
- Suzuki N, Kurata T. Studies on the repair capacity of a UV-resistant human cell strain, Uvr-1. J Radiat Res 1982;23:63.
- Leavitt J. Discovery and characterization of 2 novel human cancer-related proteins using 2-dimensional gel-electrophoresis. Electrophoresis 1994;15:345-57.
- Park TS, Chen ZP, Leavitt J. Activation of the leukocyte plastin gene occurs in most human cancer cells. Cancer Res 1994;54:1775-81.
- Leavitt J, Chen ZP, Lockwood CJ, Schatz F. Regulation of synthesis of the transformation-induced protein, leukocyte plastin, by ovarian-steroid hormones. Cancer Res 1994;54:3447-54.
- Lin CS, Lau A, Huynh T. Differential regulation of L- and T-plastin genes by steroid receptors and upstream enhancers. FASEB J 1997;11:3203.
- Zheng JP, Rudra-Ganguly N, Miller GJ, Moffatt KA, Cote RJ, Roy-Burman P. Steroid hormone induction and expression patterns of L-plastin in normal and carcinomatous prostate tissues. Am J Pathol 1997;150:2009-18.
- Zheng JP, Rudra-Ganguly N, Powell WC, Roy-Burman P. Suppression of prostate carcinoma cell invasion by expression of antisense L-plastin gene. Am J Pathol 1999;155:115-22.
- Akbulut H, Zhang LX, Tang YC, Deisseroth A. Cytotoxic effect of replication-competent adenoviral vectors carrying L-plastin promoter regulated E1A, cytosine deaminase genes in cancers of the breast, ovary and colon. Cancer Gene Ther 2003;10:388-95.
- Akbulut H, Tang YC, Maynard J, Zhang LX, Pizzorno G, Deisseroth A. Vector targeting makes 5-fluorouracil chemotherapy less toxic and more effective in animal models of epithelial neoplasms. Clin Cancer Res 2004;10:7738-46.
- Mine M, Koarada S, Sai T, Miyake K, Kimoto M. Peptide-binding motifs of the mixed haplotype A beta(z)/A alpha(d) major histocompatibility complex class II molecule: a restriction element for auto-reactive T cells in (NZBxNZW)F-1 mice. Immunology 1998;95:577-84.
- Shinomiya H, Nagai K, Hirata H, Kobayashi N, Hasegawa H, Liu FZ, et al. Preparation and characterization of recombinant murine p65/L-plastin expressed in Escherichia coli and high-titer antibodies against the protein. Biosci Biotech Biochem 2003;67:1368-75.
- Neto ECD, Kumar A, Shadick NA, Michon AM, Matsudaira P, Eaton RB, et al. Antibodies to T-isoform and L-isoform of the cytoskeletal protein, fimbrin, in patients with systemic lupus-erythematosus. J Clin Invest 1992;90:1037-42.
- Kobayashi H, Kokubo T, Abe K, Sato K, Kimura S, Miyokawa N, et al. Analysis of anchor residues in a naturally processed HCA-DR53 ligand. Immunogenetics 1996;44:366-71.
- Galiegue-Zouitina S, Quief S, Hildebrand MP, Denis C, Detourmi-gnies L, Lai JL, et al. Nonrandom fusion of L-plastin (LCPI) and LAZ3(BCL6) genes by t(3;13)(q27;q14) chromosome translocation in two cases of B-cell non-Hodgkin lymphoma. Genes Chromosomes Cancer 1999;26:97-105.
- Niaudet P. Nephrotic syndrome in children. Curr Opin Pediatr 1993;5:174-9.
- Shalhoub RJ. Pathogenesis of lipoid nephrosis. Disorder of T-cell function. Lancet 1974;2:556-60.
- Sahali D, Pawlak A, Valanciute A, Grimbert P, Lang P, Remy P, et al. A novel approach to investigation of the pathogenesis of active minimal-change nephrotic syndrome using subtracted cDNA library screening. J Am Soc Nephrol 2002;13:1238-47.
- Grimbert P, Valanciute A, Audard V, Pawlak A, Le Gouvelo S, Lang P, et al. Truncation of C-mip (Tc-mip), a new proximal signaling protein, induces c-maf Th2 transcription factor and cytoskeleton reorganization. J Exp Med 2003;198:797-807.
- Belmont LD, Drubin DG. The yeast V159N actin mutant reveals roles for actin dynamics in vivo. J Cell Biol 1998;142:1289-99.
- Timmers ACJ, Niebel A, Balague C, Dagkesaruanskaya A. Differential localisation of GFP fusions to cytoskeleton-binding proteins in animal, plant, and yeast cells. Protoplasma 2002;220:69-78.